
- Photonics Research
- Vol. 10, Issue 9, 2122 (2022)
Abstract
1. INTRODUCTION
Pulsed lasers have attracted tremendous interest owing to their excellent time-domain and frequency-domain characteristics. They have been applied in various applications, such as industrial processing, atmospheric monitoring, medical surgery, and scientific research [1–3]. The passive modulation technique is a standard method to obtain a pulsed laser, which has the advantages of low cost, simple structure, and easy implementation [4]. The critical component, the saturable absorber (SA), is synthesized based on the saturable absorption characteristics of nonlinear optical (NLO) materials. With the development of pulsed lasers for high power, short pulses, tunable operating wavelengths, and miniaturization, the demand for NLO materials with excellent comprehensive wideband performance has become more apparent [5,6].
The ideal NLO materials for SAs should have the characteristics of large nonlinearity, a wide absorption band, a fast response time, ambient atmospheric stability, high-power processing capacity, low optical loss, and low cost [7,8]. Traditional chromium-doped yttrium aluminum garnet crystals and semiconductor saturable absorber mirrors have several drawbacks that seriously limit their practical applications, especially in the mid-IR (MIR) band: narrowband operation, wavelength sensitivity, limited response time, and complex preparation processes [9–11].
In recent years, 2D materials have attracted tremendous interest because of their unique electronic and optical properties, including adjustable bandgap values and band structures, high carrier density and mobility, and ultrawide NLO responses [12–16]. Combined with their mechanical flexibility and good compatibility with optical elements, various 2D materials such as graphene, black phosphorus (BP), transition metal dichalcogenides (TMDs), and topological insulators (TIs) have been practically applied in photoelectric devices [17–22]. Among them, TMDs have the chemical formula
Sign up for Photonics Research TOC. Get the latest issue of Photonics Research delivered right to you!Sign up now
Compared to 2H-polytype TMDs, research on the NLO properties and related applications of 1T-polytype TMDs is relatively sparse. As a 1T-polytype TMD,
Figure 1.Band gap distribution of several typical 2D materials.
To date,
2. CRYSTAL GROWTH AND CHARACTERIZATION
In this study, we used an improved atmospheric pressure CVD method to prepare
Figure 2.Schematic diagram of CVD reactor.
During the reaction, the temperature inside the reactor was maintained at 850°C.
Figure 3.(a) Crystal structure of
X-ray diffraction (XRD) was used to characterize the crystallographic structure of the prepared
To explore the surface morphology of the prepared 2D nanosheets, field-emission scanning electron microscopy (FESEM; Auriga, Carl Zeiss; WD: 7.8 mm; EHT: 1.50 kV; scan mode: SE2) was performed. As shown in Fig. 3(c), typical
Transmission electron microscopy (TEM; Talos F200X, Thermo Fisher Scientific) was used to explore the structural arrangement of the prepared
To confirm the linear optical properties of the prepared
Figure 4.(a) Visible NIR transmission spectra of the
3. ULTRAFAST CARRIER DYNAMICS
To explore the ultrafast carrier dynamics of
Figure 5.(a) TAS pumped at 1500 nm and probed from 490 to 900 nm. (b) TAS pumped at 1500 nm and probed from 700 to 1100 nm. (c), (d) Extracted TAS with different delay time.
Moreover, we analyzed the intrinsic physical processes and response times of
Figure 6.(a) Attenuation dynamic curve at different wavelengths. (b) Illustration of the carrier dynamics process in
The experimental results of the recovery process can be fitted well with a tri-exponential decay model. The relationship between
Fitting Parameters of TAS Dynamics of 1T-TaS2 at Different Wavelengths
726 | 0.35 | 0.11 | 6.50 |
973 | 0.46 | 0.19 | 7.56 |
1030 | 0.57 | 0.18 | 8.78 |
Figure 6(b) shows the carrier dynamics process of
4. NLO PROPERTIES
According to the TAS experiments above, a broadband negative absorption signal was observed in the 2D
Figure 7 shows the OA Z-scan experimental results for the 2D
Figure 7.(a) Schematic of saturable absorption. Z-scan results of
The normalized transmittance along the
The insets in Figs. 7(b)–7(f) show the relationship between transmittance and input intensity. The light intensity distribution along the
Table 2 summarizes the NLO parameters of
NLO Parameters of 1T-TaS2 and Other Typical 2D SA Materials
Material | Reference | ||||
---|---|---|---|---|---|
515 | 15.4 | 1.2 | This work | ||
1030 | 6.7 | 0.2 | |||
1500 | 9.4 | 6.4 | |||
2000 | 9.6 | 27.4 | |||
2500 | 6.6 | 28.8 | |||
Graphene | 515 | −10.5 | — | 2.6 | [ |
1030 | −12.4 | — | 3.2 | ||
BP | 532 | — | — | [ | |
1060 | 15.6 | [ | |||
1030 | — | — | [ | ||
1040 | — | — | [ | ||
1030 | −0.96 | — | — | [ | |
Te/PVP | 1060 | 10.5 | [ |
5. PASSIVE Q-SWITCHING OF SOLID-STATE LASERS
To further examine the performance of the 2D
Figure 8.Passively
Figures 8(a)–8(c) exhibit the pulse characteristics versus the absorbed pump power at 1.06 μm. The threshold pump power for the Q-switched operation was 0.43 W. The maximum average power output of the pulse laser was 102 mW at an absorbed pump power of 1.10 W, and the corresponding optical-to-optical efficiency was approximately 9.3%. With an increase in the incident pump power, the pulse width decreased during repetition, while the single-pulse energy and peak power increased. The achieved shortest pulse width was 200.0 ns with a repetition frequency of 211.5 kHz. The corresponding largest pulse energy and the highest peak power are 0.48 μJ and 2.41 W, respectively. The measured laser emission spectrum is shown in Fig. 8(b), where the central wavelength is located at 1065.5 nm. At 1.34 μm, the Q-switched threshold pump power increased to 0.73 W. In the pump regions 0.73–2.03 W, stable Q-switched operation was observed. As the pump power increased further, the performance of the output pulse laser degraded, the pulse trains became chaotic, and the satellite pulse started to appear. The maximum Q-switched output power was 85 mW with an optical-to-optical efficiency of 4.2%. At the same pump power, the obtained pulse width and repetition frequency are 110.5 ns and 412.3 kHz, as shown in Fig. 8(d). The single-pulse energy and peak power were 0.21 μJ and 1.87 W, respectively, as shown in Fig. 8(e). The central wavelength of the emission spectrum for the pulse laser was 1339.2 nm, which is shown in the inset of Fig. 8(e). For the 1.94 μm passively Q-switched operation, the threshold pump power was 1.79 W, as shown in Fig. 8(g). The stable Q-switched regime could be sustained until the absorbed pump power increased to 4.11 W, corresponding to a slope efficiency of 7.9%. At this point, the maximum Q-switched output power was 323 mW. The obtained shortest pulse width was 692.5 ns, and the corresponding repetition frequency, single-pulse energy, and peak power were 83.0 kHz, 3.89 μJ, and 5.62 W, respectively. The laser emission spectrum is shown in the inset of Fig. 8(h), and the central wavelength was 1937.8 nm. Typical pulse trains and the corresponding single-pulse profiles at the three wavelengths are shown in Figs. 8(c), 8(f), and 8(i). Under the maximum output power, the pulse trains were clean and tidy at all three wavelengths with slight fluctuations and high stability, indicating the excellent performance of the
Table 3 summarizes the detailed output characteristics of the
Passive Q-switching Performance for a Solid-State Laser of 2D 1T-TaS2
Laser Crystal | Laser Properties | ||||||
---|---|---|---|---|---|---|---|
Absorbed Pump | CW Output | Q-switched Output | Pulse Width (ns) | Single-pulse | Peak Power (W) | ||
1.06 | Nd:YAG | 1.10 | 0.38 | 102 | 200 | 0.48 | 2.41 |
1.34 | 2.03 | 0.75 | 85 | 110.5 | 0.21 | 1.87 | |
1.94 | Tm:YAP | 4.11 | 1.37 | 323 | 692.5 | 3.89 | 5.62 |
As a novel broadband SA,
6. CONCLUSION
In conclusion,
References
[1] U. Keller. Recent developments in compact ultrafast lasers. Nature, 424, 831-838(2003).
[2] X. F. Wang, Y. Wang, B. B. Zhang, F. F. Zhang, Z. H. Yang, S. L. Pan. CsB4O6F: a congruent-melting deep-ultraviolet nonlinear optical material by combining superior functional units. Angew. Chem. Int. Ed., 129, 14307-14311(2017).
[3] H. W. Yu, J. Young, H. P. Wu, W. G. Zhang, J. M. Rondinelli, P. S. Halasyamani. Electronic, crystal chemistry, and nonlinear optical property relationships in the dugganite A3B3Cd2O14 family. J. Am. Chem. Soc., 138, 4984-4989(2016).
[4] R. I. Woodward, E. J. R. Kelleher. 2D saturable absorbers for fibre lasers. Appl. Sci., 5, 1440-1456(2015).
[5] A. Martinez, Z. Sun. Nanotube and graphene saturable absorbers for fibre lasers. Nat. Photonics, 7, 842-845(2013).
[6] Y. Zhang, X. Li, A. Qyyum, T. Feng, P. Guo, J. Jiang, H. Zheng. PbS nanoparticles for ultrashort pulse generation in optical communication region. Part. Part. Syst. Char., 35, 1800341(2018).
[7] J. Feng, X. Li, Z. Shi, C. Zheng, X. Li, D. Leng, Y. Wang, J. Liu, L. Zhu. 2D ductile transition metal chalcogenides (TMCs): novel high-performance Ag2S nanosheets for ultrafast photonics. Adv. Opt. Mater., 8, 1901762(2020).
[8] X. Li, J. Feng, W. Mao, F. Yin, J. Jiang. Emerging uniform Cu2O nanocubes for 251st harmonic ultrashort pulse generation. J. Mater. Chem. C, 8, 14386-14392(2020).
[9] T. T. Kajava, A. L. Gaeta. Q switching of a diode-pumped Nd: YAG laser with GaAs. Opt. Lett., 21, 1244-1246(1996).
[10] M. E. Jazi, M. D. Baghi, M. Hajimahmodzadeh, M. Soltanolkotabi. Pulsed Nd:YAG passive
[11] S. D. Liu, B. T. Zhang, J. L. He, H. W. Yang, J. L. Xu, F. Q. Liu, H. T. Huang. Diode-pumped passively
[12] M. Liu, X. Yin, E. Ulin-Avila, B. Geng, T. Zentgraf, L. Ju, F. Wang, X. Zhang. A graphene-based broadband optical modulator. Nature, 474, 64-67(2011).
[13] J. Ji, X. Song, J. Liu, Z. Yan, C. Huo, S. Zhang, M. Su, L. Liao, W. Wang, Z. Ni, Y. Hao, H. Zeng. Two-dimensional antimonene single crystals grown by van der Waals epitaxy. Nat. Commun., 7, 13352(2016).
[14] K. S. Novoselov, V. I. Fal’ko, L. Colombo, P. R. Gellert, M. G. Schwab, K. Kim. A roadmap for graphene. Nature, 490, 192-200(2012).
[15] C. Xing, Z. Xie, Z. Liang, W. Liang, T. Fan, J. S. Ponraj, S. C. Dhanabalan, D. Fan, H. Zhang. 2D nonlayered selenium nanosheets: facile synthesis, photoluminescence, and ultrafast photonics. Adv. Opt. Mater., 5, 1700884(2017).
[16] T. L. Li, R. Hao, L. L. Zhang, J. Y. Mao, F. Li, Y. P. Zhang, J. X. Fang, L. Zhang. Superior third-order nonlinearity in inorganic fullerene-like WS2 nanoparticles. Photon. Res., 8, 1881-1887(2020).
[17] B. Guo, Q. L. Xiao, S. Wang, H. Zhang. 2D layered materials: synthesis, nonlinear optical properties, and device applications. Laser Photon. Rev., 13, 1800327(2019).
[18] W. Liu, M. Liu, X. Liu, X. Wang, H. X. Deng, M. Lei, Z. Wei, Z. Wei. Recent advances of 2D materials in nonlinear photonics and fiber lasers. Adv. Opt. Mater., 8, 1901631(2020).
[19] Q. Bao, H. Zhang, Y. Wang, Z. Ni, Y. Yan, Z. X. Shen, K. P. Loh, D. Y. Tang. Atomic-layer graphene as a saturable absorber for ultrafast pulsed lasers. Adv. Funct. Mater., 19, 3077-3083(2009).
[20] M. X. Wang, J. Zhang, Z. P. Wang, C. Wang, S. van Smaalen, H. Xiao, X. Chen, C. L. Du, X. G. Xu, X. T. Tao. Broadband CrOCl saturable absorber with a spectral region extension to 10.6 μm. Adv. Opt. Mater., 8, 1901446(2020).
[21] G. Sobon. Mode-locking of fiber lasers using novel two-dimensional nanomaterials: graphene and topological insulators. Photon. Res., 3, A56-A63(2015).
[22] H. Lin, S. Fraser, M. H. Hong, M. Chhowalla, D. Li, B. H. Jia. Near-perfect microlenses based on graphene microbubbles. Adv. Photon., 2, 055001(2020).
[23] A. Autere, H. Jussila, Y. Dai, Y. Wang, H. Lipsanen, Z. Sun. Nonlinear optics with 2D layered materials. Adv. Mater., 30, 1705963(2018).
[24] L. Britnell, R. Ribeiro, A. Eckmann, R. Jalil, B. Belle, A. Mishchenko, Y.-J. Kim, R. Gorbachev, T. Georgiou, S. Morozov. Strong light-matter interactions in heterostructures of atomically thin films. Science, 340, 1311-1314(2013).
[25] Z. P. Sun, A. Martinez, F. Wang. Optical modulators with 2D layered materials. Nat. Photonics, 10, 227-238(2016).
[26] P. K. Cheng, C. Y. Tang, X. Y. Wang, L. H. Zeng, Y. H. Tsang. Passively
[27] B. H. Chen, X. Y. Zhang, K. Wu, H. Wang, J. Wang, J. P. Chen.
[28] M. Zhang, Q. Wu, F. Zhang, L. L. Chen, X. X. Jin, Y. W. Hu, Z. Zheng, H. Zhang. 2D black phosphorus saturable absorbers for ultrafast photonics. Adv. Opt. Mater., 7, 1800224(2019).
[29] R. Ang, Y. Tanaka, E. Ieki, K. Nakayama, T. Sato, L. Li, W. Lu, Y. Sun, T. Takahashi. Real-space coexistence of the melted Mott state and superconductivity in Fe-substituted 1T-TaS2. Phys. Rev. Lett., 109, 176403(2012).
[30] I. Vaskivskyi, I. A. Mihailovic, S. Brazovskii, J. Gospodaric, T. Mertelj, D. Svetin, P. Sutar, D. Mihailovic. Fast electronic resistance switching involving hidden charge density wave states. Nat. Commun., 7, 11442(2016).
[31] X. Xue, X. Wang, Y. Song, W. Mi. Electronic structure of transitional metal doped two dimensional 1T-TaS2: a first-principles study. J. Alloy. Compd., 739, 723-728(2018).
[32] R. Matsunaga, N. Tsuji, H. Fujita, A. Sugioka, K. Makise, Y. Uzawa, H. Terai, Z. Wang, H. Aoki, R. Shimano. Light-induced collective pseudospin precession resonating with Higgs mode in a superconductor. Science, 345, 1145-1149(2014).
[33] E. J. Sie, C. M. Nyby, C. D. Pemmaraju, S. J. Park, X. Shen, J. Yang, M. C. Hoffmann, B. K. Ofori-Okai, R. Li, A. H. Reid, S. Weathersby, E. Mannebach, N. Finney, D. Rhodes, D. Chenet, A. Antony, L. Balicas, J. Hone, T. P. Devereaux, T. F. Heinz, X. Wang, A. M. Lindenberg. An ultrafast symmetry switch in a Weyl semimetal. Nature, 565, 61-66(2019).
[34] T. Danz, T. Domröse, C. Ropers. Ultrafast nanoimaging of the order parameter in a structural phase transition. Science, 371, 371-374(2021).
[35] T. Patel, J. Okamoto, T. Dekker, B. W. Yang, J. J. Gao, X. Luo, W. J. Lu, Y. P. Sun, A. W. Tsen. Photocurrent imaging of multi-memristive charge density wave switching in two-dimensional 1T-TaS2. Nano Lett., 20, 7200-7206(2020).
[36] J. Martincová, M. Otyepka, P. Lazar. Oxidation of metallic two-dimensional transition metal dichalcogenides: 1T-MoS2 and 1T-TaS2. 2D Mater., 7, 045005(2020).
[37] K. Hu, Q. J. Chen, S. Y. Xie. Pressure induced superconductive 10-fold coordinated TaS2: a first-principles study. J. Phys. Condens. Matter, 32, 085402(2019).
[38] R. Zhao, Y. Wang, D. Deng, X. Luo, W. Lu, Y. Sun, Z. Liu, L. Qin, J. Robinson. Tuning phase transitions in 1T-TaS2 via the substrate. Nano Lett., 17, 3471-3477(2017).
[39] L. Perfetti, P. A. Loukakos, M. Lisowski, U. Bovensiepen, H. Berger, S. Biermann, P. S. Cornaglia, A. Georges, M. Wolf. Time evolution of the electronic structure of 1T-TaS2 through the insulator-metal transition. Phys. Rev. Lett., 97, 067402(2006).
[40] Y. Yu, F. Yang, X. Lu, Y. Yan, Y.-H. Cho, L. Ma, X. Niu, S. Kim, Y.-W. Son, D. Feng, S. Li, S. W. Cheong, X. Chen, Y. Zhang. Gate-tunable phase transitions in thin flakes of 1T-TaS2. Nat. Nanotechnol., 10, 270-276(2015).
[41] W. Fu, Y. Chen, J. H. Lin, X. W. Wang, Q. S. Zeng, J. D. Zhou, L. Zheng, H. Wang, Y. M. He, H. Y. He, Q. D. Fu, K. Suenaga, T. Yu, Z. Liu. Controlled synthesis of atomically thin 1T-TaS2 for tunable charge density wave phase transitions. Chem. Mater., 28, 7613-7618(2016).
[42] M. A. K. Pathan, A. Gupta, M. E. Vaida. Exploring the growth and oxidation of 2D-TaS2 on Cu (111). Nanotechnology, 32, 505605(2021).
[43] F. Jellinek. The system tantalum-sulfur. J. Less Common Metals, 4, 9-15(1962).
[44] P. Lazar, J. Martincová, M. Otyepka. Structure, dynamical stability, and electronic properties of phases in TaS2 from a high-level quantum mechanical calculation. Phys. Rev. B, 92, 224104(2015).
[45] Q. Dong, Q. Li, S. Li, X. Shi, S. Niu, S. Liu, R. Liu, B. Liu, X. Luo, J. Si, W. Lu, N. Hao, Y. Sun, B. Liu. Structural phase transition and superconductivity hierarchy in 1T-TaS2 under pressure up to 100 GPa. NPJ Quantum Mater., 6, 20(2021).
[46] X. S. Wang, H. N. Liu, J. X. Wu, J. H. Lin, W. He, H. Wang, X. H. Shi, K. Suenaga, L. M. Xie. Chemical growth of 1T-TaS2 monolayer and thin films: robust charge density wave transitions and high bolometric responsivity. Adv. Mater., 30, 1800074(2018).
[47] H. C. Lan, F. Liang, X. X. Jiang, C. Zhang, H. H. Yu, Z. S. Lin, H. J. Zhang, J. Y. Wang, Y. C. Wu. Pushing nonlinear optical oxides into the mid-infrared spectral region beyond 10 μm: design, synthesis, and characterization of La3SnGa5O14. J. Am. Chem. Soc., 140, 4684-4690(2018).
[48] J. W. Shi, J. R. Zhu, X. X. Wu, B. Y. Zheng, J. Chen, X. Y. Sui, S. Zhang, J. Shi, W. N. Du, Y. G. Zhong, Q. Wang, Q. Zhang, A. Pan, X. F. Liu. Enhanced trion emission and carrier dynamics in monolayer WS2 coupled with plasmonic nanocavity. Adv. Opt. Mater., 8, 2001147(2020).
[49] Q. C. Sun, L. Yadgarov, R. Rosentsveig, G. Seifert, R. Tenne, J. L. Musfeldt. Observation of a Burstein–Moss shift in rhenium-doped MoS2 nanoparticles. ACS Nano, 7, 3506-3511(2013).
[50] A. Chernikov, C. Ruppert, H. M. Hill, A. F. Rigosi, T. F. Heinz. Population inversion and giant bandgap renormalization in atomically thin WS2 layers. Nat. Photonics, 9, 466-470(2015).
[51] T. N. Lin, S. R. M. Santiago, S. P. Caigas, C. T. Yuan, T. Y. Lin, J. L. Shen, Y. F. Chen. Many-body effects in doped WS2 monolayer quantum disks at room temperature. npj 2D Mater. Appl., 3, 46(2019).
[52] H. R. Mu, Y. Liu, S. R. Bongu, X. Z. Bao, L. Li, S. Xiao, J. C. Zhuang, C. Liu, Y. Huang, Y. Dong, K. Helmerson, J. Wang, G. Y. Liu, Y. Du, Q. L. Bao. Germanium nanosheets with Dirac characteristics as a saturable absorber for ultrafast pulse generation. Adv. Mater., 33, 2101042(2021).
[53] W. T. Yan, Y. P. Han, Q. Fu, T. Xu, S. Yin, W. Wu, W. Liu. Ultrafast carrier relaxation in SnSe
[54] M. C. Downer, C. V. Shank. Ultrafast heating of silicon on sapphire by femtosecond optical pulses. Phys. Rev. Lett., 56, 761-764(1986).
[55] M. B. Pushkarsky, I. G. Dunayevskiy, M. Prasanna, A. G. Tsekoun, R. Go, C. K. N. Patel. High-sensitivity detection of TNT. Proc. Natl. Acad. Sci. USA, 103, 19630-19634(2006).
[56] R. Ikuta, Y. Kusaka, T. Kitano, H. Kato, T. Yamamoto, M. Koashi. Wide-band quantum interface for visible-to-telecommunication wavelength conversion. Nat. Commun., 2, 537(2011).
[57] L. Zhou, Y. Wang, Y. Wang, S. Xiao, J. He. Saturable absorption and self-defocusing response of 2D monoelemental germanium nanosheets in broadband spectra. Opt. Express, 29, 39115-39124(2021).
[58] M. Sheik-Bahae, A. A. Said, T. H. Wei, D. J. Hagan, E. W. Van Stryland. Sensitive measurement of optical nonlinearities using a single beam. IEEE J. Quantum Electron., 26, 760-769(1990).
[59] E. Garmire. Resonant optical nonlinearities in semiconductors. IEEE J. Sel. Top. Quantum Electron., 6, 1094-1110(2000).
[60] J. Guo, J. L. Zhao, D. Z. Huang, Y. Z. Wang, F. Zhang, Y. Q. Ge, Y. F. Song, C. Y. Xing, D. Y. Fa, H. Zhang. Two-dimensional tellurium-polymer membrane for ultrafast photonics. Nanoscale, 11, 6235-6242(2019).
[61] S. F. Zhang, C. R. Shen, I. M. Kislyakov, N. N. Dong, A. Ryzhov, X. Y. Zhang, I. M. Belousova, J. M. Nunzi, J. Wang. Photonic-crystal-based broadband graphene saturable absorber. Opt. Lett., 44, 4785-4788(2019).
[62] Y. W. Wang, G. H. Huang, H. R. Mu, S. H. Lin, J. Z. Chen, S. Xiao, Q. L. Bao, J. He. Ultrafast recovery time and broadband saturable absorption properties of black phosphorus suspension. Appl. Phys. Lett., 107, 091905(2015).
[63] T. Wang, X. X. Jin, J. Yang, J. Wu, Q. Yu, Z. H. Pan, H. S. Wu, J. Z. Li, R. T. Su, J. M. Xu, K. Zhang, T. C. He, P. Zhou. Ultra-stable pulse generation in ytterbium-doped fiber laser based on black phosphorus. Nanoscale Adv., 1, 195-202(2019).
[64] S. Zhang, N. Dong, N. McEvoy, M. O’Brien, S. Winters, N. C. Berner, C. Yim, Y. Li, X. Zhang, Z. Chen, L. Zhang, G. S. Duesberg, J. Wang. Direct observation of degenerate two-photon absorption and its saturation in WS2 and MoS2 monolayer and few-layer films. ACS Nano, 9, 7142-7150(2015).
[65] N. N. Dong, Y. X. Li, S. F. Zhang, N. McEvoy, X. Y. Zhang, Y. Cui, L. Zhang, G. S. Duesberg, J. Wang. Dispersion of nonlinear refractive index in layered WS2 and WSe2 semiconductor films induced by two-photon absorption. Opt. Lett., 41, 3936-3939(2016).
[66] R. N. Verrone, C. Moisset, F. Lemarchand, A. Campos, M. Univ, C. P. Pellegrino, J. L. Lumeau, J. Y. Natoli, K. Iliopoulos. Thickness-dependent optical nonlinearities of nanometer-thick Sb2Te3 thin films: implications for mode-locking and super-resolved direct laser writing. ACS Appl. Nano Mater., 3, 7963-7972(2020).
[67] P. D. Cunningham, A. T. Hanbicki, K. M. McCreary, B. T. Jonker. Photoinduced bandgap renormalization and exciton binding energy reduction in WS2. ACS Nano, 11, 12601-12608(2017).
[68] Y. Li, X. Wu, W. Liu, H. Xu, X. Liu. Revealing the interrelation between C- and A-exciton dynamics in monolayer WS2 via transient absorption spectroscopy. Appl. Phys. Lett., 119, 051106(2021).
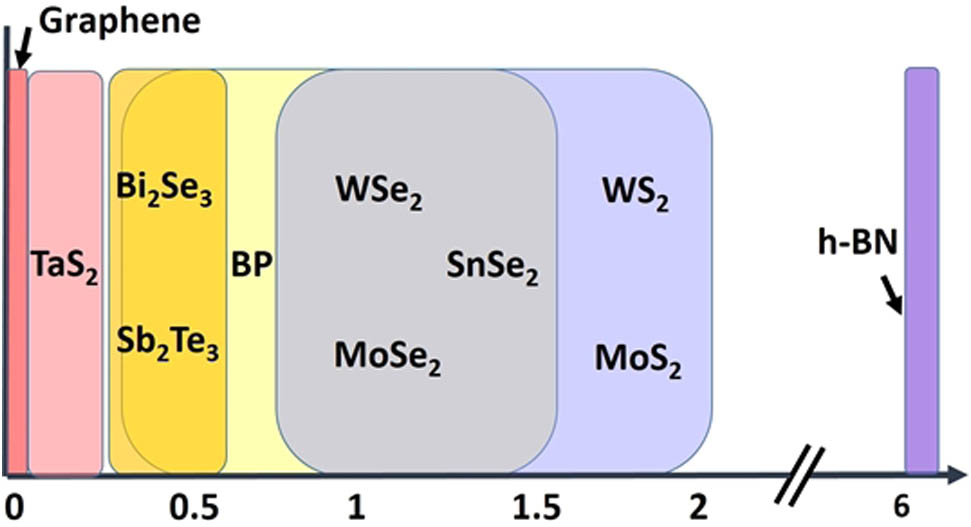
Set citation alerts for the article
Please enter your email address