
- Photonics Research
- Vol. 10, Issue 3, 786 (2022)
Abstract
1. INTRODUCTION
The rapid manipulation of the flow of light in photonic microstructures has been increasingly investigated in nano-optics and nanophotonics [1–4]. Thanks to the excellent tunability, multistimuli-responsiveness, and easy fabrication [5], photonic devices based on soft self-assembly materials have gained significant attention [6,7], whereas one of the key challenges is related to the unsatisfactory response time. Liquid crystals (LCs), as excellent self-assembly building blocks, have developed many highly functional and planar optical systems, including smart windows, structured light generators, and optical communications [8–11]. To date, many efforts have been carried out in attempting to improve the response time. For instance, the simple ordered structure and proper physical anisotropies make nematic LC indefectible in display industries and other light modulation applications [12–14]. LC molecules respond to the electric field by reorienting the director, i.e., the average molecular orientation, parallel or perpendicular to the field [12,15,16]. Once the electric field is removed, the director relaxes back to the ground state with a typical response time of approximately several milliseconds [16]. Recently, nanosecond switching of nematic LC was reported, based on the electrical modification of the order parameters [17–19]. Additionally, distinct LC microstructures with different physical properties and driving schemes, such as dual-frequency LCs [20], polymer dispersed LCs [21], and ferroelectric LCs [22], have been widely investigated to speed up operations. However, few related studies have investigated the fast switching of chiral LCs with photonic microstructures.
Chiral LCs are well known as versatile materials with Bragg reflection [23–25]. They can self-assemble into periodic helical structures with a helical pitch
Figure 1.Optical characteristics of CLCs. (a) Schematic illustration of the 1D helical structure. Blue rods represent LC directors. White bidirectional arrow denotes the alignment direction. Green and pink arrows indicate the transmission and reflection of light. (b) POM images of planar CLCs at different
Here, we demonstrate the fast electro-optic switching of CLCs composed of a negative nematic host and a chiral dopant. The location of the PBG is optimized by tuning the concentration
Sign up for Photonics Research TOC. Get the latest issue of Photonics Research delivered right to you!Sign up now
2. RESULTS AND DISCUSSIONS
The CLC mixtures are composed of a host nematic LC with a negative dielectric anisotropy and a guest chiral dopant. When mixtures are infiltrated into a unidirectionally aligned cell, a self-assembled 1D helical structure with planar texture is formed [Figs. 1(a) and 1(b)]. Here, we define the alignment direction as the
The PBG of CLC can be tuned by changing
The temperature-dependencies of parameters
To explore the fast electro-optic responses of CLC, we use a left-handed circularly polarized laser beam (
Figure 2.Electro-optic responses of CLC. (a) Dynamics of the transmitted light intensity of CLC under
To further examine the mechanism of the submicrosecond response of soft photonic crystal materials, we investigate related electric effects during the switching process. Due to the complexity of CLC with continuously varied director orientations along the helical axis, LC molecules, which are arranged in a quasi-nematic manner (Fig. 3), within a localized layer are extracted to provide a clearer picture of the optical tensor modification. In the absence of the electric field, LC molecules show a uniaxial orientational order with the optical tensor
Figure 3.Schematic illustration of field-induced effects. (a) Orientational order of LC molecules and ellipsoid optical tensor in the absence of an electric field. (b) Thermal fluctuation of the optical tensor. (c) Orientational order of LC molecules and elongated oblate optical tensor under an electric field. (d) Quenching of the optical tensor fluctuation.
To distinguish the main contribution to the fast switching of CLC, we utilize three experimental geometries [18] (see the Materials and Methods section). In geometry BU, the field-induced effective birefringence change
Figure 4.Three experimental geometries. (a) Geometry BU:
Then, we investigate the corresponding field-induced birefringence change. Detailed experimental methods are described in the Materials and Methods section. As a result, the
Figure 5.Submicrosecond electro-optic switching of the nematic LC host. (a) Field-induced
3. CONCLUSIONS
In conclusion, we demonstrate submicrosecond electro-optic switching of a soft chiral photonic crystal material that is composed of a negative nematic LC host and a chiral dopant. The addition of the chiral dopant triggers the self-assembly of a helical configuration, where the chirality is transferred from the molecular nanoscale to the structural microscale. The concentration of chiral dopant is optimized so that one of the PBG edges matches the wavelength of the probe laser beam. Due to the negative dielectric anisotropy of the LC host, the vertical electric field does not reorient the LC directors but modifies the orientational order parameter of LC molecules. Distinct from the switching principle in nematic LCs [17–19], the refractive index change of CLCs causes the shift of PBG, which alters the forbidden state of photons and, consequently, causes the modulation of light transmittance. The electro-optic response of CLCs is also compared with that of the nematic LC host, which presents a close response time under the same
The field-induced refractive index change is approximately 0.01, which leads to a small shift of PBG around the wavelength of 632.8 nm. Fortunately, by adopting nematic hosts with larger birefringence, the edge shift of PBG can be substantially increased, thus enhancing the light modulation. By doping a photoresponsive additive into CLCs, the position of PBG can be modulated by union control of light exposure and electric field [37–39]. Thus, the range of the working wavelength can be largely extended. The fast switching of CLCs is based on the shift of PBG with a circular polarization selectivity, which allows unique highly functional photonic devices beyond the modulation of the optical phase retardation. In addition, one can devise many more ways of manipulating the flow of light by suitably combining the rapid switching mechanism of CLCs with special optical elements, such as polarization gratings, microlasers, Pancharatnam–Berry lenses, metasurfaces, and fibers.
4. MATERIALS AND METHODS
Materials: CLC mixtures were composed of a nematic LC host HNG715600-100 (Jiangsu Hecheng Display Technology) and the guest chiral dopant S811, (S)-2-octyl 4-[4-(hexyloxy)benzoyloxy]benzoate (Merck KGaA) with various concentrations. The dielectric anisotropy of HNG715600-100 is
Sample fabrication: Glass substrates (
Characterizations: The reflection spectra of CLCs were measured with unpolarized light (Tungsten halogen lamp LS-1, Ocean Optics), a polarizer, a spectrometer (USB4000 UV-VIS, Ocean Optics), and a hot stage (PE94, Linkam). The nanosecond DC voltage pulses were generated by a pulse generator (HV 1000, Direct Energy) with sharp rise and fall edges with the characteristic time of 1 ns. A circularly polarized laser beam was generated by a He–Ne laser (
Experimental geometries: The
Acknowledgment
Acknowledgment. The authors gratefully appreciate Prof. Oleg D. Lavrentovich for his constructive discussions.
References
[1] Y. Zhou, Z. Yuan, X. Gong, M. Birowosuto, C. Dang, Y. C. Chen. Dynamic photonic barcodes for molecular detection based on cavity-enhanced energy transfer. Adv. Photon., 2, 066002(2020).
[2] Y. Wang, Q. Chen, W. Yang, Z. Ji, L. Jin, X. Ma, Q. Song, A. Boltasseva, J. Han, V. M. Shalaev, S. Xiao. High-efficiency broadband achromatic metalens for near-IR biological imaging window. Nat. Commun., 12, 5560(2021).
[3] J. Xiong, S. T. Wu. Planar liquid crystal polarization optics for augmented reality and virtual reality: from fundamentals to applications. eLight, 1, 3(2021).
[4] Y. Xiong, F. Xu. “Multifunctional integration on optical fiber tips: challenges and opportunities. Adv. Photon., 2, 064001(2020).
[5] J. Hu, W. Wang, H. Yu. Endowing soft photo-actuators with intelligence. Adv. Intell. Syst., 1, 1900050(2019).
[6] M. Kolle, S. Lee. Progress and opportunities in soft photonics and biologically inspired optics. Adv. Mater., 30, 1702669(2018).
[7] C. Ropp, N. Bachelard, D. Barth, Y. Wang, X. Zhang. Dissipative self-organization in optical space. Nat. Photonics, 12, 739-743(2018).
[8] L. Wang, H. K. Bisoyi, Z. Zheng, K. G. Gutierrez-Cuevas, G. Singh, S. Kumar, T. J. Bunning, Q. Li. Stimuli-directed self-organized chiral superstructures for adaptive windows enabled by mesogen-functionalized graphene. Mater. Today, 20, 230-237(2017).
[9] L. Qin, W. Gu, J. Wei, Y. Yu. Piecewise phototuning of self-organized helical superstructures. Adv. Mater., 30, 1704941(2018).
[10] Y. Liu, H. Liang, C. W. Chen, X. Xie, W. Hu, P. Chen, J. Wen, J. Zhou, T. H. Lin, I. C. Khoo. Ultrafast switching of optical singularity eigenstates with compact integrable liquid crystal structures. Opt. Express, 26, 28818-28826(2018).
[11] D. Chen, M. R. Tuchband, B. Horanyi, E. Korblova, D. M. Walba, M. A. Glaser, J. E. Maclennan, N. A. Clark. Diastereomeric liquid crystal domains at the mesoscale. Nat. Commun., 6, 7763(2015).
[12] C. G. Pochi Yeh. Optics of Liquid Crystal Displays(1999).
[13] Q. H. Wang, F. Chu, H. Dou, L. L. Tian, R. Li, W. Y. Hou. A single-cell-gap transflective liquid crystal display with a vertically aligned cell. Liq. Cryst., 46, 1183-1190(2018).
[14] Y. H. Lin, Y. J. Wang, H. C. Lin, M. L. Lee, P. L. Chen. Optical measurement in a curved optical medium with optical birefringence and anisotropic absorption. Opt. Express, 29, 38654-38668(2021).
[15] Z. Shen, S. Zhou, X. Li, S. Ge, P. Chen, W. Hu, Y. Lu. Liquid crystal integrated metalens with tunable chromatic aberration. Adv. Photon., 2, 036002(2020).
[16] D. K. Yang, S. T. Wu. Fundamentals of Liquid Crystal Devices(2014).
[17] V. Borshch, S. V. Shiyanovskii, O. D. Lavrentovich. Nanosecond electro-optic switching of a liquid crystal. Phys. Rev. Lett., 111, 107802(2013).
[18] V. Borshch, S. V. Shiyanovskii, B. X. Li, O. D. Lavrentovich. Nanosecond electro-optics of a nematic liquid crystal with negative dielectric anisotropy. Phys. Rev. E, 90, 062504(2014).
[19] B. X. Li, V. Borshch, S. V. Shiyanovskii, S. B. Liu, O. D. Lavrentovich. Electro-optic switching of dielectrically negative nematic through nanosecond electric modification of order parameter. Appl. Phys. Lett., 104, 201105(2014).
[20] Y. H. Fan, H. Ren, X. Liang, Y. H. Lin, S. T. Wu. Dual-frequency liquid crystal gels with submillisecond response time. Appl. Phys. Lett., 85, 2451-2453(2004).
[21] S. Pagidi, R. Manda, S. S. Bhattacharyya, S. G. Lee, S. M. Song, Y. J. Lim, J. H. Lee, S. H. Lee. Fast switchable micro-lenticular lens arrays using highly transparent nano-polymer dispersed liquid crystals. Adv. Mater. Interfaces, 6, 1900841(2019).
[22] Q. Guo, K. Yan, V. Chigrinov, H. Zhao, M. Tribelsky. Ferroelectric liquid crystals: physics and applications. Crystals, 9, 470(2019).
[23] Z. G. Zheng, Y. Li, H. K. Bisoyi, L. Wang, T. J. Bunning, Q. Li. Three-dimensional control of the helical axis of a chiral nematic liquid crystal by light. Nature, 531, 352-356(2016).
[24] W. Hu, H. Zhao, L. Song, Z. Yang, H. Cao, Z. Cheng, Q. Liu, H. Yang. Electrically controllable selective reflection of chiral nematic liquid crystal/chiral ionic liquid composites. Adv. Mater., 22, 468-472(2010).
[25] L. J. Chen, L. L. Gong, Y. L. Lin, X. Y. Jin, H. Y. Li, S. S. Li, K. J. Che, Z. P. Cai, C. J. Yang. Microfluidic fabrication of cholesteric liquid crystal core–shell structures toward magnetically transportable microlasers. Lab Chip, 16, 1206-1213(2016).
[26] J. Li, H. K. Bisoyi, J. Tian, J. Guo, Q. Li. Optically rewritable transparent liquid crystal displays enabled by light-driven chiral fluorescent molecular switches. Adv. Mater., 31, 1807751(2019).
[27] J. Xiang, Y. Li, Q. Li, D. A. Paterson, J. M. D. Storey, C. T. Imrie, O. D. Lavrentovich. Electrically tunable selective reflection of light from ultraviolet to visible and infrared by heliconical cholesterics. Adv. Mater., 27, 3014-3018(2015).
[28] Y. Li, S. Huang, P. Zhou, S. Liu, J. Lu, X. Li, Y. Su. Polymer-stabilized blue phase liquid crystals for photonic applications. Adv. Mater. Technol., 1, 1600102(2016).
[29] H. Coles, S. Morris. Liquid-crystal lasers. Nat. Photonics, 4, 676-685(2010).
[30] D. J. Mulder, A. P. H. J. Schenning, C. W. M. Bastiaansen. Chiral-nematic liquid crystals as one dimensional photonic materials in optical sensors. J. Mater. Chem. C, 2, 6695-6705(2014).
[31] P. Chen, L. L. Ma, W. Hu, Z. X. Shen, H. K. Bisoyi, S. B. Wu, S. J. Ge, Q. Li, Y. Q. Lu. Chirality invertible superstructure mediated active planar optics. Nat. Commun., 10, 2518(2019).
[32] W. S. Li, L. L. Ma, L. L. Gong, S. S. Li, C. Yang, B. Luo, W. Hu, L. J. Chen. Interlaced cholesteric liquid crystal fingerprint textures via sequential UV-induced polymer-stabilization. Opt. Mater. Express, 6, 19-28(2016).
[33] K. H. Kim, D. H. Song, Z. G. Shen, B. W. Park, K. H. Park, J. H. Lee, T. H. Yoon. Fast switching of long-pitch cholesteric liquid crystal device. Opt. Express, 19, 10174-10179(2011).
[34] X. Shang, L. Meeus, D. Cuypers, H. De Smet. Fast switching cholesteric liquid crystal optical beam deflector with polarization independence. Sci. Rep., 7, 6492(2017).
[35] S. W. Oh, T. H. Yoon. Fast bistable switching of a cholesteric liquid crystal device induced by application of an in-plane electric field. Appl. Opt., 53, 7321-7324(2014).
[36] H. Yoshida, Y. Inoue, Y. Shiozaki, M. Takahashi, H. Kubo, A. Fujii, M. Ozaki. Fast and continuous tunable lasing from a nano-pore embedded cholesteric liquid crystal film. Mol. Cryst. Liq. Cryst., 560, 101-107(2012).
[37] H. K. Bisoyi, T. J. Bunning, Q. Li. Stimuli-driven control of the helical axis of self-organized soft helical superstructures. Adv. Mater., 30, 1706512(2018).
[38] K. G. Gutierrez-Cuevas, L. Wang, Z. G. Zheng, H. K. Bisoyi, G. Li, L. S. Tan, R. A. Vaia, Q. Li. Frequency-driven self-organized helical superstructures loaded with mesogen-grafted silica nanoparticles. Angew. Chem. Int. Ed., 55, 13090-13094(2016).
[39] C. L. Yuan, W. Huang, Z. G. Zheng, B. Liu, H. K. Bisoyi, Y. Li, D. Shen, Y. Lu, Q. Li. Stimulated transformation of soft helix among helicoidal, heliconical, and their inverse helices. Sci. Adv., 5, eaax9501(2019).
[40] B. X. Li, G. Babakhanova, R. L. Xiao, V. Borshch, S. Siemianowski, S. V. Shiyanovskii, O. D. Lavrentovich. Microsecond electro-optic switching of nematic liquid crystals with giant dielectric anisotropy. Phys. Rev. Appl., 12, 024005(2019).
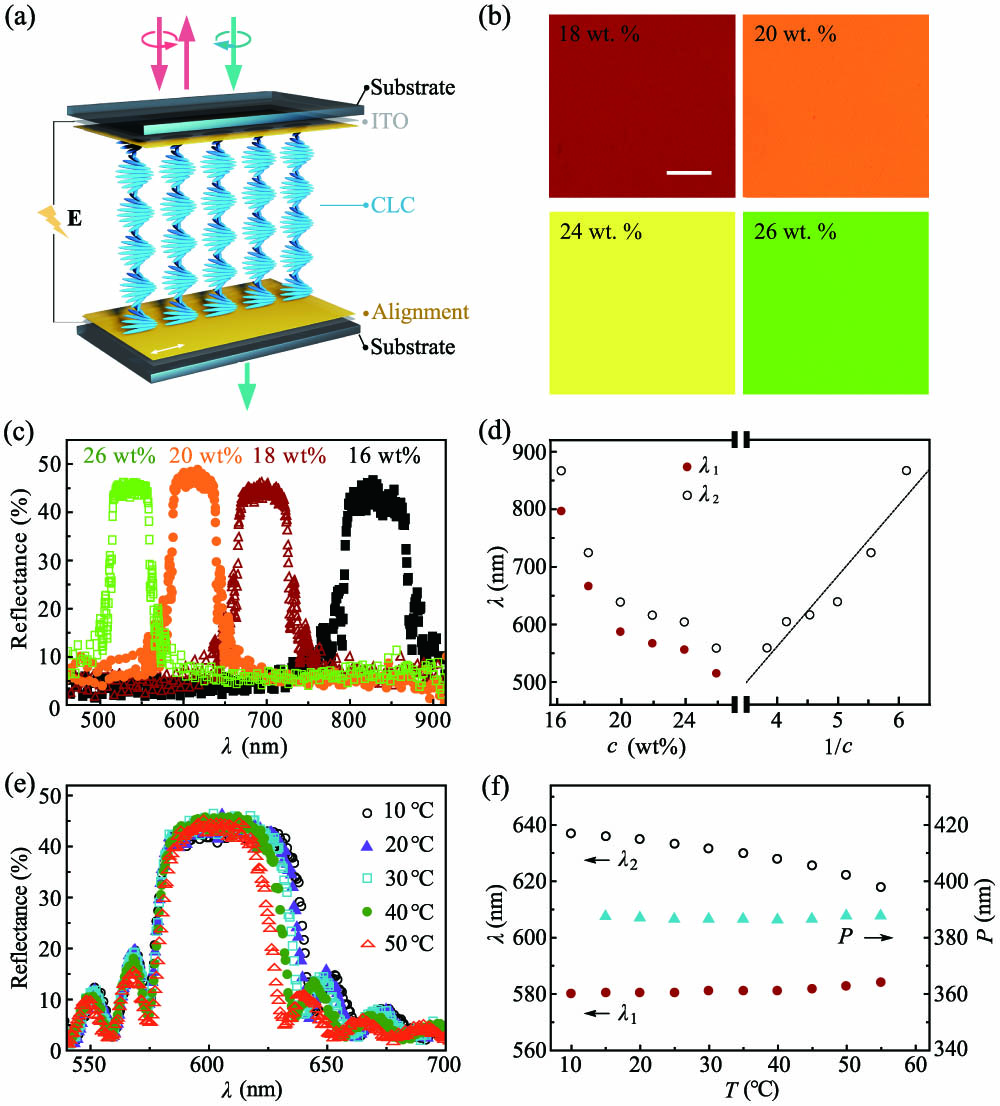
Set citation alerts for the article
Please enter your email address