Qiangqiang Wang1、†, Jiqing Tan1, Qi Jie1, Hongxing Dong2、*, Yongsheng Hu1, Chun Zhou2, Saifeng Zhang3, Yichi Zhong2, Shuang Liang1、4, Long Zhang2、5, Wei Xie1、6、*, and Hongxing Xu1、4、*
Author Affiliations
1East China Normal University, School of Physics and Electronic Science, State Key Laboratory of Precision Spectroscopy, Shanghai, China2Chinese Academy of Sciences, Shanghai Institute of Optics and Fine Mechanics, Key Laboratory of Materials for High-Power Laser, Shanghai, China3Shanghai University, Department of Physics, Shanghai, China4Wuhan University, School of Physics and Technology, Center for Nanoscience and Nanotechnology, Wuhan, China5University of Chinese Academy of Sciences, Hangzhou Institute for Advanced Study, Hangzhou, China6Chongqing Institute of East China Normal University, Chongqing Key Laboratory of Precision Optics, Chongqing, Chinashow less
DOI: 10.1117/1.AP.5.5.055001
Cite this Article
Set citation alerts
Qiangqiang Wang, Jiqing Tan, Qi Jie, Hongxing Dong, Yongsheng Hu, Chun Zhou, Saifeng Zhang, Yichi Zhong, Shuang Liang, Long Zhang, Wei Xie, Hongxing Xu. Perturbation-driven echo-like superfluorescence in perovskite superlattices[J]. Advanced Photonics, 2023, 5(5): 055001
Copy Citation Text
show less
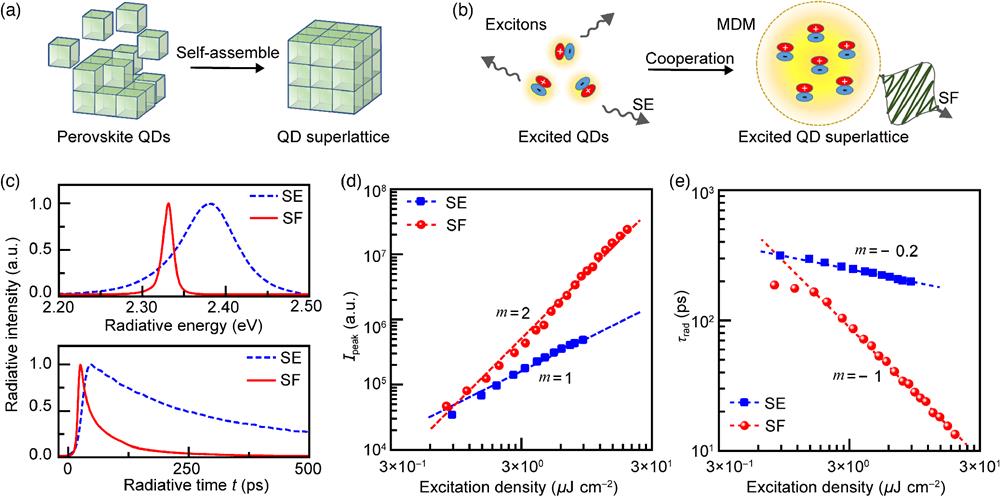
Fig. 1. SF effect in perovskite QD superlattice. (a) Sketch of a superlattice sample assembled by QDs. The size of the individual cubic QDs is , and the size of the assembled superlattices is distributed from submicrometers to micrometers. (b) Physical pictures of the excited states and the different radiation effects in corresponding samples. An exciton is shown as a pair of “±,” and the MDM is a collective state of a dipole ensemble with an MDM and a synchronous radiation phase. The yellow halo around the “±” pair presents the virtual light field. Dense excitons in a QD superlattice share the virtual light fields and from MDM. Black curved arrows describe the substantial radiation fields, i.e., the SE from individual excitons and the SF from cooperative excitons. (c) Time-integrated and time-resolved spectra. The SE signals from individual QDs and the SF signals from an assembled superlattice are measured under excitation densities of 6.1 and per pulse, respectively. (d), (e) Excitation density versus the time-resolved peak intensity and the radiation decay time . The dashed lines are guidelines for the trends . and are obtained by fitting the time-resolved spectra under different excitation densities.
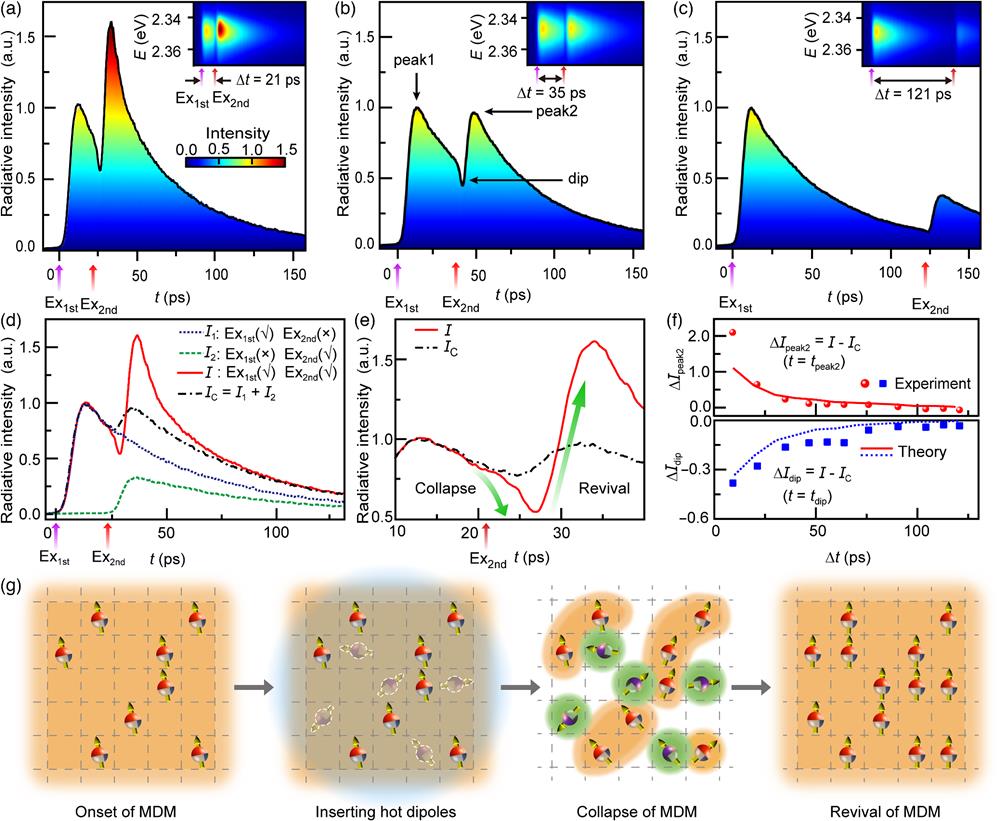
Fig. 2. Echo-like SF behavior under a controllable disturbance. (a)–(c) Time-resolved photoluminescence (PL) spectra at 10 K. The intensities are normalized by the intensity of the first peak. The arrows below the horizontal axis indicate the pulsed excitation times. The pulse densities and are fixed at 5.4 and , respectively. The insets show the radiation energy/time-resolved mapping data. The row data at the spectral peak center are extracted and plotted in the corresponding main graph. (d) Comparison of the experimental results (, , ) and the comparison data (). The excitation parameters are the same as those in (a). (e) Zooming in the echo-like part in (d). (f) Disturbance-induced intensity variations (, ) versus the disturbance injection moment (). (g) Physical explanation of the echo-like radiation. The red (purple) spheres represent excitons pumped by (). The brown arrows passing across spheres describe the cooperative radiation phase. The blue halo represents the laser field of , which adds new hot excitons to the previous cooperative exciton ensemble. The orange (green) background is the virtual light field shared by the cooperative (hot) excitons. The grid lines represent the QD units in the superlattice sample.
Fig. 3. Echo-like SF behavior versus the temperature of crystal lattice. (a) Temperature-dependent cooperation state of the exciton ensemble, which is determined by the competition of two mechanisms, i.e., the cooperative mechanism via the virtual light field (represented by orange/green background) and the dephasing mechanism via phonon scattering (represented by the twisted lattice). The state of the exciton ensemble changes from “cooperative” at 10 K to “partially cooperative” at 50 K and “noncooperative” at 100 K. (b) Radiation response for an exciton ensemble at different temperatures. The data shown by solid lines are excited by and with a fixed pulse density of , and an interval time of . The data shown by dashed lines are excited by only.
Fig. 4. Echo-like SF behavior versus the disturbance strength. (a) Radiation dynamics for different disturbance amplitudes. Other excitation parameters (, , and ) are fixed. All curves are normalized to the intensity of the first peak, and each curve is equally spaced along the vertical axis for clarity. (b) Comparison of three disturbance cases. The dip region (dashed box) is further magnified. (c) Echo-like SF versus disturbance amplitude. The radiation intensities without and with the disturbance are shown as black and colored lines, respectively. The largest dip occurs at a moderate disturbance amplitude , depending on the competition between scattering dephasing and the rebuilding rate of the MDM.