
- Journal of Semiconductors
- Vol. 40, Issue 7, 071903 (2019)
Abstract
1. Introduction
Different from the classical light source, the single photon emitter (SPE) is a fundamental quantum light resource for many scalable quantum technologies including quantum computing, quantum precision measurement and quantum secure communication. The ideal on-demand SPE emits exactly one photon at a time into a given spatiotemporal mode, and all photons are identical so that if any two are sent through separate arms of a beam-splitter, they produce full interference that is a signature of indistinguish ability. Up to now, researchers have found SPEs in zero-dimensional materials, such as GaAs, InGaAs QDs[
Recently, the SPEs from defect states have been found in various 2D materials, including TMDs MX2 (WSe2, WS2, MoSe2, and MoS2)[
The previous reviews discussed the advances in mastering SPEs in 2D materials[
2. Quantum emitters from various 2D materials
In the family of 2D materials, TMDs aroused great interest because of their excellent optical properties[
Fig. 1(a) shows the photoluminescence (PL) intensity mapping of narrow emission lines centered at 1.719 eV in monolayer WSe2, where the triangle indicates the position of the monolayer WSe2. The emissions from defects in WSe2 are expressed by bright spots appeared at three isolated positions, they are different from the emission of intrinsic excitons. Fig. 1(b) shows the PL spectrum of one isolated position in Fig. 1(a), the right inset shows the intrinsic excitons of monolayer WSe2, and the left inset shows one single quantum emitter with doublet peak structure. The narrow linewidth is the most remarkable characteristic of single photon emission in the spectrum. In terms of emission energy, the emission position of defects is red shifted by 40–100 meV from the intrinsic excitons transition of monolayer WSe2, this shows that the energy of the quantum light source is within the bandgap of WSe2. To further confirm the doublet peak is single photon emission, the corresponding second order correlation function was measured, from the fitting data in Fig. 1(c), the
Figure 1.(Color online) (a) Photoluminescence (PL) intensity map of narrow emission lines centered at 1.719 eV. The dashed triangle indicates the position of the monolayer WSe2[
Quantum emitters based on layered semiconductor GaSe was also reported[
The temperature is important for the quantum emitters based devices. 2D material hBN is a promising candidate for new generation single photon sources due to its chemical stability, and large bandgap with about 6 eV of monolayer hBN[
3. Electrically driven quantum light in a WSe2-based light-emitting diode
With the development of technology, nanodevices have been widely used in many fields. In application, most of them are driven by electricity. Electrically driven quantum light source, as the core devices in the field of quantum communication and quantum computing, have attracted more and more attentions[
Recently, Carmen et al. reported the electrically driven quantum light in a WSe2-based LED[
Figure 2.(Color online) (a) Optical microscope image of a typical device used in experiments. The dotted lines highlight the footprint of the graphene, hBN and the TMD layers individually. The Cr/Au electrodes contact the graphene and TMD layers to provide electrical bias[
Fig. 2(b) shows the spatial map of integrated Electro-luminescence (EL) from the WSe2-based LED device at 10 K. The spatially uniform light emissions are from delocalized excitons of WSe2. The brighter area corresponds to the bilayer WSe2, suggesting that most of the injected current flows through this region. The dotted circles correspond to highly localized emissions from both the monolayer and the bilayer WSe2. The device is controlled by an applied voltage. At zero bias between the graphene and the monolayer WSe2, the Fermi energy (EF) of the system is the same across the heterostructure. Therefore, this preventing net charge flow (current) between the layers. When a negative bias applied to the graphene electrode, as shown in Fig. 2(c), the graphene EF raises to above the minimum of the conduction band (EC) of grounded WSe2, this induces electrons to tunnel from the graphene into the monolayer WSe2. The radiative recombination between the tunneled electrons and the holes results in the photoemission. When WS2 monolayer exfoliated from an n-doped bulk crystal is integrated into the same device, it can also be electrically driven to emit single photons of different wavelengths[
The emissions from TMDs based electrically driven quantum light device also have good quantum qualities. Fig. 2(d) shows the example emissions spectrum from mono- and bilayers WSe2, emission in monolayer and bilayer WSe2 are described by the top and bottom spectrum, respectively. In the spectrum, shaded area highlights the emission due to intrinsic excitons of WSe2, whereas quantum LED (QLED) produces spectra at longer wavelength for both mono- and bilayers of WSe2, because localized states are also within the bandgap of WSe2. In bilayers WSe2, its quantum emission peak is shifted to longer wavelength compared with in monolayer. If different layers of TMDs are integrated into this device, it can be used as a means to adjust the wavelength of single photon emission.
EL has unique characteristics compared with PL. As shown in Fig. 2(e), when excitons states are excited by electricity at a low-current regime, the localized excitons states respond more efficiently to charge injection than the delocalized ones at 10 K. At room temperature, the emission wavelength of EL shifts to long wavelength due to the charge injection. Fig. 2(f) plots the current dependence of the integrated EL intensity from a quantum emitter, as well as from the unbound monolayer WSe2 excitons. The saturation behavior of QLED intensity with the increase of current intensity is observed. The corresponding second order correlation function of QLED was measured at low temperature, as shown in Fig. 2(g). The
4. Tailoring the quantum emitters by nanopillars and strain engineering
2D materials have atomic-level thickness, hence the quantum emitter in 2D material is sensitive to the environment and applied field such as magnetic field, localized plasmonic field as well as strain field. For instance, magnetic fields can dramatically tune the optical spectra of SPEs WSe2 due to its large g factor of ~8.7[
By placing the 2D material on spatially ordered nanopillars, the position of single photon emission can be controlled. A simple method is to create array nanopillars and then covered with monolayer 2D materials[
Figure 3.(Color online) (a) Scanning electron microscope (SEM) image of nanopillar substrate, fabricated by electron beam lithography[
Strain engineering, another strain controlled method for single photon emission in 2D materials, has good application prospects. By using nanoscale elastic strain engineering in WSe2, it can deterministically generate robust quantum emitters by apply local modification of the electronic and optical properties[
5. The entanglement between phonons and single photon
The strain not only can tune the quantum emission, but also can tune the phonon vibration through lattice deformation. This rises an opportunity to tune the defect-phonon coupling and quantum entanglement between photon and phonon[
Fig. 4(a) shows the basic experimental configuration. The monolayer WSe2/hBN stack connected with metal electrodes, which can be regarded as a field effect transistor device. By tuning the voltage, different energy of emission can be obtained from QDs in monolayer WSe2. Fig. 4(b) shows the basic idea behind entanglement of a CP with an photon. In previous studies, CP in WSe2 has been reported[
Figure 4.(Color online) (a) Optical image of the monolayer WSe2/hBN stack. The dashed square indicates the scanning area in the PL mapping measurements[
Fig. 4(c) shows the cross sectional PL spectra at a fixed Vg. The parent emission peaks marked by “a” can be classified some groups according to their energy. The splitting peaks marked by “b” correspond to entanglement process. Moreover, the energy spacing between “a” and “b” peaks, for all the three QDs is precisely 21.8 meV. This energy spacing is exactly equal to the measured energy of the
The polarization properties of the doublets are essential factor to further understand of detail of entanglement. Fig. 4(d) shows that the red and blue peaks of the D3a parent peak are linearly polarized and orthogonal to each other. This is consistent with the D3a doublet’s assignment as a localized X0. However, the b peaks, in spite of being phonon replicas and inheriting all their properties from the parent peaks, show completely unpolarized emission in both linear and circular basis measurements as shown in Fig. 4(e). Considering the mixed state of polarization,
6. Conclusion and outlook
As the holders of quantum emitters, 2D materials have great advantages, one of which is that the extraction efficiency of luminescence can reach near unity because it is no longer reflected back to materials due to the atomic thickness. TMDs, as an important member of 2D material family, have good optical properties, and show great potential as quantum emitters in many studies. But their bandgap determines that in most cases they can only emit stable and distinguishable single photons at low temperatures. As another 2D material with wide bandgap, hBN can overcome the problem that single photon cannot be emitted at room temperature, even at higher temperature. Together with tunable emission wavelength and high brightness, hBN will be one of best candidate for room temperature single photon source. However, there is still much challenge to prepare mono- and few-layer 2D materials samples with large area and high quality. Mechanical exfoliation method can obtain 2D materials with good quality, but the size is small and the yield is low. Chemical vapor deposition can be used to synthesize many 2D materials, but it often has high requirements for substrates. At the same time, in the process of material synthesis by chemical method, the quality is usually not good enough. Therefore, it is necessary to make defects controllable in 2D materials for improving the performance of single photon devices. Recently, Liu’s group in Peaking University reported epitaxial growth of a 100-square-centimetre single-crystal hexagonal boron nitride monolayer on copper substrate[
2D Van der Waals heterostructure assembled by different 2D materials can also be easily coupled with other devices such as photonic crystal and plasmonic nanocavity. It provides many opportunities to tune the properties of quantum emitters. In particular, by tuning the angle between bilayer TMDs heterostructures, the interlayer Moiré exciton can be generated at certain twist angle owing to the exciton trapped by Moiré periodic potential[
The physical origin of single photon emission is unclear up to date. Once the origin of defect sates in 2D materials is known, the single photon emitter or SPE array could be easily produced on demand by ion implanting or chemical doping. By coupling 2D materials on the nanopillars, it is easy to generate quantum emitter array by strain effects, but uniformity and stability of different emitter pixel need to be improved for quantum application. The quantum entanglement between single photon and chiral phonon opens up the feasibility of controlling single photon emission by phonons. This also provides a good foundation for the development of quantum optomechanical devices in 2D materials.
Acknowledgments
We acknowledge support from the National Basic Research Program of China (Grant No. 2017YFA0303401, 2016YFA0301200), Beijing Natural Science Foundation (JQ18014), and Strategic Priority Research Program of Chinese Academy of Sciences (Grant No. XDB28000000).
References
[1] J Hours, S Varoutsis, M Gallart et al. Single photon emission from individual GaAs quantum dots. Appl Phys Lett, 82, 2206(2003).
[2] E Stock, T Warming, I Ostapenko et al. Single-photon emission from InGaAs quantum dots grown on (111) GaAs. Appl Phys Lett, 96, 145(2010).
[3] D Dalacu, P J Poole, R L Williams. Nanowire-based sources of non-classical light. Nanotechnology, 30, 232001(2019).
[4] X Ma, N F Hartmann, J K Baldwin et al. Room-temperature single-photon generation from solitary dopants of carbon nanotubes. Nate Nanotechnol, 10, 671(2015).
[5] M Arita, F Le Roux, M J Holmes et al. Ultraclean single photon emission from a GaN quantum dot. Nano Lett, 17, 2902(2017).
[6] I Aharonovich, E Neu. Diamond nanophotonics. Adv Opt Mater, 2, 911(2014).
[7] N Elke, H Christian, H Michael et al. Low-temperature investigations of single silicon vacancy colour centres in diamond. New J Phys, 15, 043005(2013).
[8] I Aharonovich, C Zhou, A Stacey et al. Enhanced single-photon emission in the near infrared from a diamond color center. Phys Rev B, 79, 1377(2009).
[9] S Manzeli, D Ovchinnikov, D Pasquier et al. 2D transition metal dichalcogenides. Nat Rev Mater, 2, 17033(2017).
[10] A Srivastava, M Sidler, A V Allain et al. Optically active quantum dots in monolayer WSe2. Nat Nanotechnol, 10, 491(2015).
[11] C Chakraborty, K M Goodfellow, V A Nick. Localized emission from defects in MoSe2 layers. Opt Mater Express, 6, 2081(2016).
[12] C Cong, J Shang, Y Wang, T Yu. Optical properties of 2D semiconductor WS2. Adv Opt Mater, 6, 1700767(2018).
[13] H M Hill, A F Rigosi, C Roquelet et al. Observation of excitonic rydberg states in monolayer MoS2 and WS2 by photoluminescence excitation spectroscopy. Nano Lett, 15, 2992(2015).
[14] M Koperski, K Nogajewski, A Arora et al. Single photon emitters in exfoliated WSe2 structures. Nat Nanotechnol, 10, 503(2015).
[15] C Chakraborty, L Kinnischtzke, K M Goodfellow et al. Voltage-controlled quantum light from an atomically thin semiconductor. Nat Nanotechnol, 10, 507(2015).
[16] Y Ye, X Dou, K Ding et al. Single photon emission from deep-level defects in monolayer WSe2. Phys Rev B, 95, 245313(2017).
[17] J D Qiao, F H Mei, Y Ye. Single-photon emitters in van der Waals materials. Chin Opt Lett, 17, 020011(2019).
[18] Y M He, G Clark, J R Schaibley et al. Single quantum emitters in monolayer semiconductors. Nat Nanotechnol, 10, 497(2015).
[19] P Tonndorf, S Schwarz, J Kern et al. Single-photon emitters in GaSe. 2D Mater, 4, 021010(2017).
[20] N R Jungwirth, B Calderon, Y Ji et al. Temperature dependence of wavelength selectable zero-phonon emission from single defects in hexagonal boron nitride. Nano Lett, 16, 6052(2016).
[21] T T Tran, C Zachreson, A M Berhane et al. Quantum emission from defects in single-crystalline hexagonal boron nitride. Phys Rev Appl, 5, 034005(2016).
[22] B Sontheimer, M Braun, N Nikolay et al. Photodynamics of quantum emitters in hexagonal boron nitride revealed by low-temperature spectroscopy. Phys Rev B, 96, 121202(2017).
[23] Z Shotan, H Jayakumar, C R Considine et al. Photoinduced modification of single-photon emitters in hexagonal boron nitride. ACS Photonics, 3, 2490(2016).
[24] A W Schell, T T Tran, H Takashima et al. Non-linear excitation of quantum emitters in hexagonal boron nitride multiplayers. APL Photonics, 1, 091302(2016).
[25] R Bourrellier, S Meuret, A Tararan et al. Bright UV single photon emission at point defects in h-BN. Nano Lett, 16, 4317(2016).
[26] M Kianinia, B Regan, S A Tawfik et al. Robust solid-state quantum system operating at 800 K. ACS Photonics, 4, 768(2017).
[27] A L Exarhos, D A Hopper, R N Patel et al. Magnetic-field-dependent quantum emission in hexagonal boron nitride at room temperature. Nat Commun, 10, 222(2019).
[28] C Palacios-Berraquero, D M Kara, A R P Montblanch et al. Large-scale quantum-emitter arrays in atomically thin semiconductors. Nat Commun, 8, 15093(2017).
[29] A Branny, S Kumar, R Proux et al. Deterministic strain-induced arrays of quantum emitters in a two-dimensional semiconductor. Nat Commun, 8, 15053(2017).
[30] Y Xue, H Wang, Q Tan et al. Anomalous pressure characteristics of defects in hexagonal boron nitride flakes. ACS Nano, 12, 7127(2018).
[31] J E Kennard, J P Hadden, L Marseglia et al. On-chip manipulation of single photons from a diamond defect. Phys Rev Lett, 111, 213603(2013).
[32] T T Tran, D Wang, Z Q Xu et al. Deterministic coupling of quantum emitters in 2D materials to plasmonic nanocavity arrays. Nano Lett, 17, 2634(2017).
[33] I Aharonovich, D Englund, M Toth. Solid-state single-photon emitters. Nat Photonics, 10, 631(2016).
[34] F Xia, H Wang, D Xiao et al. Two-dimensional material nanophotonics. Nat Photonics, 8, 899(2014).
[35] R Lv, J A Robinson, R E Schaak et al. Transition metal dichalcogenides and beyond: synthesis, properties, and applications of single- and few-layer nanosheets. Accounts Chem Res, 48, 56(2015).
[36] T T Tran, C Elbadawi, D Totonjian et al. Robust multicolor single photon emission from point defects in hexagonal boron nitride. ACS Nano, 10, 7331(2016).
[37] K Watanabe, T Taniguchi, H Kanda. Direct-bandgap properties and evidence for ultraviolet lasing of hexagonal boron nitride single crystal. Nat Mater, 3, 404(2004).
[38] Q H Wang, K Kalantar-Zadeh, A Kis et al. Electronics and optoelectronics of two-dimensional transition metal dichalcogenides. Nat Nanotechnol, 7, 699(2012).
[39] Z Yuan, B E Kardynal, R M Stevenson et al. Electrically driven single-photon source. Science, 295, 102(2002).
[40]
[41] S Schwarz, A Kozikov, F Withers et al. Electrically pumped single-defect light emitters in WSe2. 2D Mater, 3, 025038(2016).
[42] C Palacios-Berraquero, M Barbone, D M Kara et al. Atomically thin quantum light-emitting diodes. Nat Commun, 7, 12978(2016).
[43] I Hapke-Wurst, U Zeitler, R J Haug et al. Mapping the
[44] G Grosso, H Moon, B Lienhard et al. Tunable and high-purity room temperature single-photon emission from atomic defects in hexagonal boron nitride. Nat Commun, 8, 705(2017).
[45] J Kern, I Niehues, P Tonndorf et al. Nanoscale positioning of single-photon emitters in atomically Thin WSe2. Adv Mater, 28, 7101(2016).
[46] M Aspelmeyer, T J Kippenberg, F Marquardt. Cavity optomechanics. Rev Mod Phys, 86, 1391(2014).
[47] M J Burek, J D Cohen, S M Meenehan et al. Diamond optomechanical crystals. Optica, 3, 1404(2016).
[48] K V Kepesidis, S D Bennett, S Portolan et al. Phonon cooling and lasing with nitrogen-vacancy centers in diamond. Phys Rev B, 88, 064105(2013).
[49] E Togan, Y Chu, A S Trifonov et al. Quantum entanglement between an optical photon and a solid-state spin qubit. Nature, 466, 730(2010).
[50] X Xu, W Yao, D Xiao et al. Spin and pseudospins in layered transition metal dichalcogenides. Nat Phys, 10, 343(2014).
[51] X Chen, X Lu, S Dubey et al. Entanglement of single-photons and chiral phonons in atomically thin WSe2. Nat Phys, 15, 221(2018).
[52] H Y Zhu, J Yi, M Y Li et al. Observation of chiral phonons. Science, 359, 579(2018).
[53] L Wang, X Xu, L Zhang et al. Epitaxial growth of a 100-square-centimetre single-crystal hexagonal boron nitride monolayer on copper. Nature, 570, 91(2019).
[54] K Tran, G Moody, F Wu et al. Evidence for moire excitons in van der Waals heterostructures. Nature, 567, 71(2019).
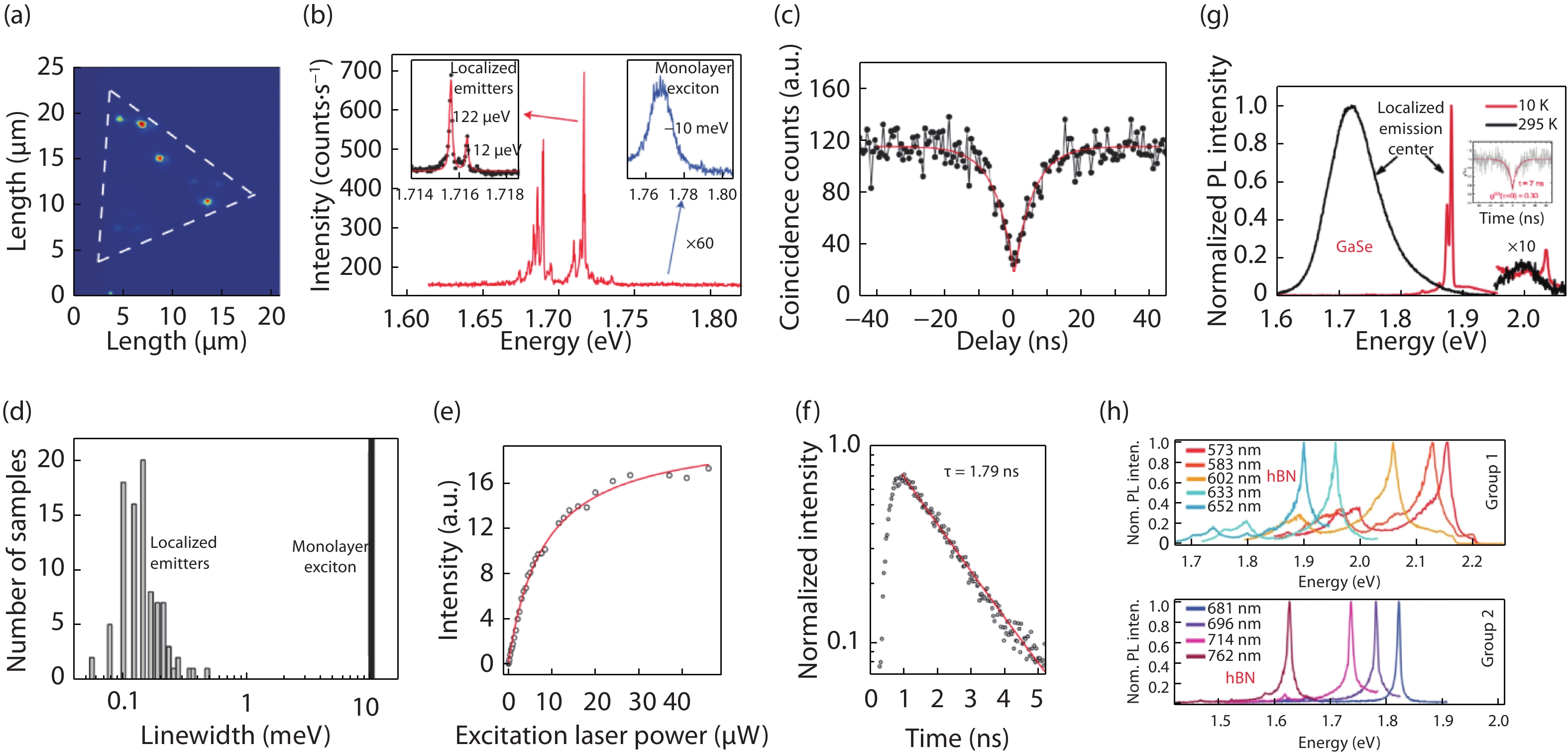
Set citation alerts for the article
Please enter your email address