H. H. Ma1、2、3、*, X. F. Li1、2、4, S. M. Weng1、2, S. H. Yew1、2, S. Kawata3, P. Gibbon4、5, Z. M. Sheng1、2、6、7, and J. Zhang1、2
Author Affiliations
1Key Laboratory for Laser Plasmas (MoE), School of Physics and Astronomy, Shanghai Jiao Tong University, Shanghai 200240, China2Collaborative Innovation Center of IFSA, Shanghai Jiao Tong University, Shanghai 200240, China3Graduate School of Engineering, Utsunomiya University, Yohtoh 7-1-2, Utsunomiya 321-8585, Japan4Institute for Advanced Simulation, Jülich Supercomputing Centre, Forschungszentrum Jülich, 52425 Jülich, Germany5Centre for Mathematical Plasma Astrophysics, Katholieke Universiteit Leuven, 3000 Leuven, Belgium6SUPA, Department of Physics, University of Strathclyde, Glasgow G4 0NG, United Kingdom7Tsung-Dao Lee Institute, Shanghai Jiao Tong University, Shanghai 200240, Chinashow less
Fig. 1. Properties of a sunlight-like laser pulse with a flat-top frequency spectrum and bandwidth Δω/ω0 = 1%. The sunlight-like laser is modeled by Eqs. (1)–(3), where the central frequency ω0 = 2πc/λ with λ = 351 nm. (a) and (b) Electric field components Ey and Ez, respectively, where the time-averaged electric field amplitude E0=〈Ey2(t)〉=〈Ez2(t)〉. (c) Amplitude frequency spectrum f(ω) and phase frequency spectrum ϕ(ω) of Ey. (d) Stokes parameters of the sunlight-like laser pulse, where I denotes the intensity regardless of polarization, Q the linear polarization along the y (+) or z (−) axis, U the linear polarization at + 45° (+) or −45° (−) from the y axis, and V the right-handed (+) or left-handed (−) circular polarization.38
Fig. 2. Properties of temporal speckles in a sunlight-like laser pulse. (a) Electric fields of two typical adjacent speckles (denoted by “S1” and “S2”) corresponding to Ey within 0.3–0.7 ps in Fig. 1(a). (b) Amplitude spectra fS1(ω) and fS2(ω) of these two speckles, showing the obvious frequency shift δω between them. (c) Statistical mean frequency shift 〈δω〉 between the central frequencies of two adjacent speckles as a function of bandwidth. (d) Statistical mean duration 〈δt〉 of a single speckle as a function of bandwidth. The statistical analyses in (c) and (d) are performed over all adjacent speckles for 〈δω〉 and over all speckles for 〈δt〉, with 100 independent broadband laser beams for each bandwidth. The statistical results under different spectral resolutions are compared by using three different laser pulse durations: 6000T0 (7.02 ps), 12 000T0 (14.04 ps), and 18 000T0 (21.06 ps).
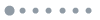
Fig. 3. (a) Average SRS reflectivity within 6000λ/c obtained from 1D PIC simulations as a function of laser intensity for a monochromatic laser (MCL), a broadband laser (BBL) with bandwidths Δω/ω0 = 1% and 2%, and a sunlight-like laser (SLL) with bandwidth Δω/ω0 = 1%, respectively. The purple dashed line indicates RSRS = 1%. (b) First saturation time of the backward-reflected light as a function of laser intensity for the three different kinds of laser beams. (c) Time evolution of the electric field of the backward laser light, where all incident lasers have the same intensity of I0 = 3 × 1015 W/cm2, and both the broadband laser and sunlight-like laser have a bandwidth Δω/ω0 = 1%. (d) Electron energy spectra for the monochromatic laser, broadband laser, and sunlight-like laser at the final simulation time of 6000λ/c. (e) and (f) Time evolution of |Ex| for the broadband laser and sunlight-like laser, respectively. In (b) and (d), all lasers have the same intensity I0 = 1.4 × 1015 W/cm2, and the broadband laser and sunlight-like laser have the same bandwidth Δω/ω0 = 1%.
Fig. 4. Wave-vector distributions of the plasma wave field Ex at 1500T0 found from 2D PIC simulations for (a) a monochromatic laser (MCL), (b) a broadband laser (BBL), and (c) a sunlight-like laser (SLL). All possible plasma wave vectors are distributed around the theoretical dashed circles owing to the wave-vector and frequency matching conditions: k0 = ks + kp, ω0 = ωs + ωp, where the subscripts 0, s, and p refer to the incident, scattered, and plasma waves, respectively. (d) Corresponding electron energy spectra at the final time of 1500T0. All the lasers have the same intensity I0 = 1.4 × 1015 W/cm2, and the broadband laser and sunlight-like laser have the same bandwidth Δω/ω0 = 1%. For simplicity, the laser intensity is assumed to be uniform in the y direction.