
- Chinese Optics Letters
- Vol. 19, Issue 11, 110602 (2021)
Abstract
1. Introduction
Ultraviolet (UV) communication has attracted the attention of many researchers in recent years, especially in the wavelength range of 200–280 nm, which is called UV-C[
However, it is considered that the UV-C light will be strongly scattered because of its short wavelength, which leads to serious light attenuation along the direction of light emission[
In recent years, temporal ghost imaging (TGI) has attracted the attention of many researchers as a modulation algorithm that can reduce the requirement of spatial and temporal resolution of detectors[
Sign up for Chinese Optics Letters TOC. Get the latest issue of Chinese Optics Letters delivered right to you!Sign up now
In this paper, we introduce the TGI algorithm to the UV-C communication system through experiments for the first time, to the best of our knowledge. It was verified that a high-frequency temporal signal could be detected by a low-bandwidth detector based on TGI in a UV-C communication system. We realized the detection and reconstruction of a 4 GHz temporal signal when the system’s bandwidth was less than 100 MHz. Meanwhile, it is proven that the TGI can realize the optimization of SNR. All of the results show that the TGI algorithm has great application potential in the UV-C communication field.
2. Methods and Experiments
In computational ghost imaging, modulation is achieved by multiplying the signal with a stack of random binary illuminated patterns in measurements. A second-order correlation between a stack of known illuminated patterns and the integration of the information received by the detector can retrieve the original signal[
Here, we choose the Hadamard matrix as
The second-order correlation between the received information and the modulation sequences is applied to retrieve the original signal[
Here,
Figures 1(a) and 1(b) show the schematic diagram of the experimental setup for the UV-C LED-based optical communication system and its picture, respectively. The original signal is 128-bit pseudo-random binary sequence (PRBS) code. The modulation of the original time signal was realized in MATLAB. After that, the transmitted signal was generated by an arbitrary waveform generator (AWG 710B, 4.2 GHz) and was loaded into the UV-C LED with a central wavelength of 277 nm as an AC component. The power supply provided a DC component to ensure that the LED worked at high emission power. The components of DC and AC were coupled through bias-tee (Mini-Circuits ZFBT-6GW+), and the superimposed electrical signal was used to drive the UV-C LED to transmit the optical signal. Then, the parameters were adjusted to ensure that the LED worked in the linear region. The transmitted optical signal passed through free space and was detected by the photodetector (Hamamatsu C12702-11, 100 MHz). At the receiving end, the received electrical signal was displayed on the oscilloscope in real-time, and, after being sampled, the LabVIEW offline program was applied to demodulate the sampled signal and evaluate the transmission performance. The transmitting frequency was adjusted on the AWG from 200 MHz to 4 GHz.
Figure 1.(a) Schematic diagram for UV-C LED-based wireless optical communication system. (b) The photograph of the experimental setup.
3. Results and Discussion
The I-V characteristic of the UV-C LED is shown in Fig. 2(a). The best working point of the UV-C LED was determined to be 30 mA for the DC signal and 1.6 V peak-to-peak voltage (
Figure 2.(a) I-V characteristics of UV-C LED. Inset is the normalized spectrum of UV-C LED. (b) Frequency characteristics of the whole system at the optimum operating point.
To describe the transmission performance of the system, we introduce the concept of PL, which is defined as[
Figure 3.(a) Comparison of transmitting frequency between OOK and TGI. (b) The calculated SNRs of OOK and TGI. Three points A, B, and C in (a) correspond to (b).
To quantitatively analyze the reason why the TGI curve drops, we calculate the SNRs of the received signals in Fig. 3(a). In general, SNR is defined as
In a word, although high-frequency signal waveforms are severely attenuated due to a lack of bandwidth, the relative amplitude between the original bits is still not significantly affected, so high-frequency signal transmission of GHz can be successfully achieved. However, when the background noise intensity is constant, the SNR at the receiving end is impacted by the dual effects of power attenuation caused by PL and waveform distortion caused by bandwidth limitation. When the attenuation and distortion are severe, the sum of information can no longer be distinguished from the background noise, and the error-free transmission cannot be realized. Therefore, when the SNR is extremely low, the error-free transmission frequency is reduced.
Moreover, the eye diagrams of the A-B-C points in Fig. 3(a) are shown in Figs. 4(a)–4(c), respectively. Comparing Fig. 4(a) with Fig. 4(b), the maximum signal distortion at the sampling instant in the eye diagram of TGI is smaller than that of OOK, and the noise margin is higher than that of OOK. Both indicate that transmission based on TGI has much weaker noise and less intersymbol interference. When the PL is the same, the influence on SNR mainly comes from the bandwidth limitation, so theoretically the eye diagram quality of point B should be worse. On the contrary, the performance of point B is significantly better than that of point A, which effectively proves that the TGI algorithm can optimize SNR. Besides, the difference between these two eye diagrams means that 200 MHz signal transmission through OOK has basically reached the upper limit of the system transmission capacity, while error-free transmission frequency based on the TGI algorithm can be further improved when the PL value is lower than 12.79 dB (point B). Comparing Fig. 4(b) with Fig. 4(c), with the increase of PL, the quality of the eye diagram decreases obviously. Different from the case when the PL value is less than 12.79 dB, the maximum error-free transmission frequency cannot be further increased because of the reduced SNR in the curve descending stage. Comparing Fig. 4(a) with Fig. 4(c), transmission frequency and PL of TGI are both larger than those of OOK, which means that the SNR of TGI is worse than that of OOK. Nevertheless, the eye diagram of TGI still looks cleaner and more regular than that of OOK, which confirms that the TGI algorithm can optimize SNR again. The above analysis of eye diagrams is consistent with that of the calculated SNRs of TGI, which are significantly higher than that of OOK in Fig. 3(b).
Figure 4.Eye diagrams comparison among (a) point A, (b) point B, and (c) point C.
The above results show that TGI can weaken the impact of bandwidth limitation to realize high-frequency signal transmission with a low-bandwidth system and simultaneously can effectively improve SNR. These advantages signify that TGI is a potential method to be applied in the field of UV-C communication with low SNR.
Besides, the quantitative analysis for transmission performance under different transmitting frequencies is also necessary. In Fig. 5(a), TGI enables error-free transmission at all frequencies. For OOK, the BER is higher than the forward error correction (FEC) threshold (
Figure 5.(a) BER comparison of OOK and TGI (PL = 0 dB). (b) The MSE of TGI at different transmitting frequencies.
4. Conclusion
In conclusion, we first introduced the TGI algorithm to UV-C communication experimentally and further demonstrated the principles, performance, and potential of it in the field of UV-C communication. TGI was used in the UV-C LED communication system to successfully transmit a 4 GHz signal whose frequency was nearly 40 times above the system bandwidth, and, simultaneously, this transmitting frequency was nearly an order of magnitude higher than that of OOK under the same circumstances. Moreover, the experimental results also show that TGI can effectively optimize the communication performance by improving the SNR. Our research lays a foundation for the application of the TGI algorithm in the UV communication domain and also provides a reference for its prolonged application in other optical communication fields.
References
[1] C. H. Kang, I. Dursun, G. Liu, L. Sinatra, X. Sun, M. Kong, J. Pan, P. Maity, E.-N. Ooi, T. K. Ng, O. F. Mohammed, O. M. Bakr, B. S. Ooi. High-speed colour-converting photodetector with all-inorganic CsPbBr3 perovskite nanocrystals for ultraviolet light communication. Light: Sci. Appl., 8, 94(2019).
[2] G. Chen, Z. Xu, H. Ding, B. M. Sadler. Path loss modeling and performance trade-off study for short-range non-line-of-sight ultraviolet communications. Opt. Express, 17, 3929(2009).
[3] X. He, E. Xie, M. S. Islim, A. A. Purwita, J. J. D. McKendry, E. Gu, H. Haas, M. D. Dawson. 1 Gbps free-space deep-ultraviolet communications based on III-nitride micro-LEDs emitting at 262 nm. Photon. Res., 7, B41(2019).
[4] Y. Tang, G. Q. Ni, Z. L. Wu, L. J. Zhang, Y. Lin. Research on channel character of solar blind UV communication. Proc. SPIE, 6829, 682907(2007).
[5] M. A. El-Shimy, S. Hranilovic. Spatial-diversity imaging receivers for non-line-of-sight solar-blind UV communications. J. Lightwave Technol., 33, 2246(2015).
[6] D. Han, Y. Liu, K. Zhang, P. Luo, M. Zhang. Theoretical and experimental research on diversity reception technology in NLOS UV communication system. Opt. Express, 20, 15833(2012).
[7] T. Shan, J. Ma, T. Wu, Z. Shen, P. Su. Single scattering turbulence model based on the division of effective scattering volume for ultraviolet communication. Chin. Opt. Lett., 18, 120602(2020).
[8] X. Zhou, X. Tan, Y. Wang, X. Song, T. Han, J. Li, W. Lu, G. Gu, S. Liang, Y. Lü, Z. Feng. High-performance 4H-SiC p-i-n ultraviolet avalanche photodiodes with large active area. Chin. Opt. Lett., 17, 090401(2019).
[9] Z. Xu, B. M. Sadler. Ultraviolet communications: potential and state-of-the-art. IEEE Commun. Mag., 46, 67(2008).
[10] S. Karp, R. M. Gagliardi, S. E. Moran, L. B. Stotts. Optical Channels(1988).
[11] G. A. Shaw, A. M. Siegel, J. Model. Extending the range and performance of non-line-of-sight ultraviolet communication links. Proc. SPIE, 6231, 62310C(2006).
[12] A. K. Majumdar, Q. He, C. C. Davis, B. M. Sadler, Z. Xu. Modulation and coding tradeoffs for non-line-of-sight ultraviolet communications. Proc. SPIE, 7464, 74640H(2009).
[13] D.-Y. Peng, J. Shi, G.-H. Peng, S.-L. Xiao, S.-H. Xu, S. Wang, F. Liu. An ultraviolet laser communication system using frequency-shift keying modulation scheme. Optoelectron. Lett., 11, 65(2015).
[14] P. Luo, M. Zhang, D. Han, Q. Li. Performance analysis of short-range NLOS UV communication system using Monte Carlo simulation based on measured channel parameters. Opt. Express, 20, 23489(2012).
[15] M. Noshad, M. Brandt-Pearce, S. G. Wilson. NLOS UV communications using M-ary spectral-amplitude-coding. IEEE Trans. Commun., 61, 1544(2013).
[16] C. Xu, H. Zhang. Packet error rate analysis of IM/DD systems for ultraviolet scattering communications. IEEE Military Communications Conference(2015).
[17] Y. Wang, S. Gu. Ultraviolet communication system based on BPSK subcarrier intensity modulation. Proc. SPIE, 9446, 94461K(2015).
[18] . https://www.acgih.org/science/tlv-bei-guidelines/.
[19] O. Alkhazragi, F. Hu, P. Zou, Y. Ha, C. H. Kang, Y. Mao, T. K. Ng, N. Chi, B. S. Ooi. Gbit/s ultraviolet-C diffuse-line-of-sight communication based on probabilistically shaped DMT and diversity reception. Opt. Express, 28, 9111(2020).
[20] H. Qin, Y. Zuo, D. Zhang, Y. Li, J. Wu. Received response based heuristic LDPC code for short-range non-line-of-sight ultraviolet communication. Opt. Express, 25, 5018(2017).
[21] H. Qin, Y. Zuo, F. Li, R. Cong, L. Meng, J. Wu. Noncoplanar geometry for mobile NLOS MIMO ultraviolet communication with linear complexity signal detection. IEEE Photon. J., 9, 7906012(2017).
[22] L. Guo, D. Meng, K. Liu, X. Mu, W. Feng, D. Han. Experimental research on the MRC diversity reception algorithm for UV communication. Appl. Opt., 54, 5050(2015).
[23] K. Kojima, Y. Yoshida, M. Shiraiwa, Y. Awaji, A. Kanno, N. Yamamoto, S. Chichibu. 1.6-Gbps LED-based ultraviolet communication at 280 nm in direct sunlight. 2018 European Conference on Optical Communication (ECOC)(2018).
[24] H. Yin, H. Jia, H. Zhang, X. Wang, S. Chang, J. Yang. Extending the data rate of non-line-of-sight UV communication with polarization modulation. Proc. SPIE, 8540, 85400I(2012).
[25] E. Xie, M. Stonehouse, R. Ferreira, J. J. D. McKendry, J. Herrnsdorf, X. He, S. Rajbhandari, H. Chun, A. V. N. Jalajakumari, O. Almer, G. Faulkner, I. M. Watson, E. Gu, R. Henderson, D. O’Brien, M. D. Dawson. Design, fabrication, and application of GaN-based micro-LED arrays with individual addressing by N-electrodes. IEEE Photon. J., 9, 7907811(2017).
[27] B. Sun, M. P. Edgar, R. Bowman, L. E. Vittert, S. Welsh, A. Bowman, M. J. Padgett. 3D computational imaging with single-pixel detectors. Science, 340, 844(2013).
[28] F. Devaux, P.-A. Moreau, S. Denis, E. Lantz. Computational temporal ghost imaging. Optica, 3, 698(2016).
[29] Y.-K. Xu, S.-H. Sun, W.-T. Liu, G.-Z. Tang, J.-Y. Liu, P.-X. Chen. Detecting fast signals beyond bandwidth of detectors based on computational temporal ghost imaging. Opt. Express, 26, 99(2018).
[30] W. Meng, D. Shi, K. Yuan, L. Zha, J. Huang, Y. Wang, C. Fan. Fourier-temporal ghost imaging. Opt. Lasers Eng., 134, 106294(2020).
[31] Y. Wang, H. Chen, W. Jiang, X. Li, X. Chen, X. Meng, P. Tian, B. Sun. Optical encryption for visible light communication based on temporal ghost imaging with a micro-LED. Opt. Lasers Eng., 134, 106290(2020).
[32] Y. Tian, H. Ge, X.-J. Zhang, X.-Y. Xu, M.-H. Lu, Y. Jing, Y.-F. Chen. Acoustic ghost imaging in the time domain. Phys. Rev. Appl., 13, 064044(2020).
[33] P. Ryczkowski, M. Barbier, A. T. Friberg, J. M. Dudley, G. Genty. Ghost imaging in the time domain. Nat. Photon., 10, 167(2016).
[34] C. Zhang, S. Guo, J. Cao, J. Guan, F. Gao. Object reconstitution using pseudo-inverse for ghost imaging. Opt. Express, 22, 30063(2014).
[35] X. Sun, W. Cai, O. Alkhazragi, E.-N. Ooi, H. He, A. Chaaban, C. Shen, M. H. Oubei, M. Z. M. Khan, T. K. Ng, M.-S. Alouini, B. S. Ooi. 375-nm ultraviolet-laser based non-line-of-sight underwater optical communication. Opt. Express, 26, 12870(2018).
[36] X. Chen, M. Jin, H. Chen, Y. Wang, P. Qiu, X. Cui, B. Sun, P. Tian. Computational temporal ghost imaging for long-distance underwater wireless optical communication. Opt. Lett., 46, 1938(2021).
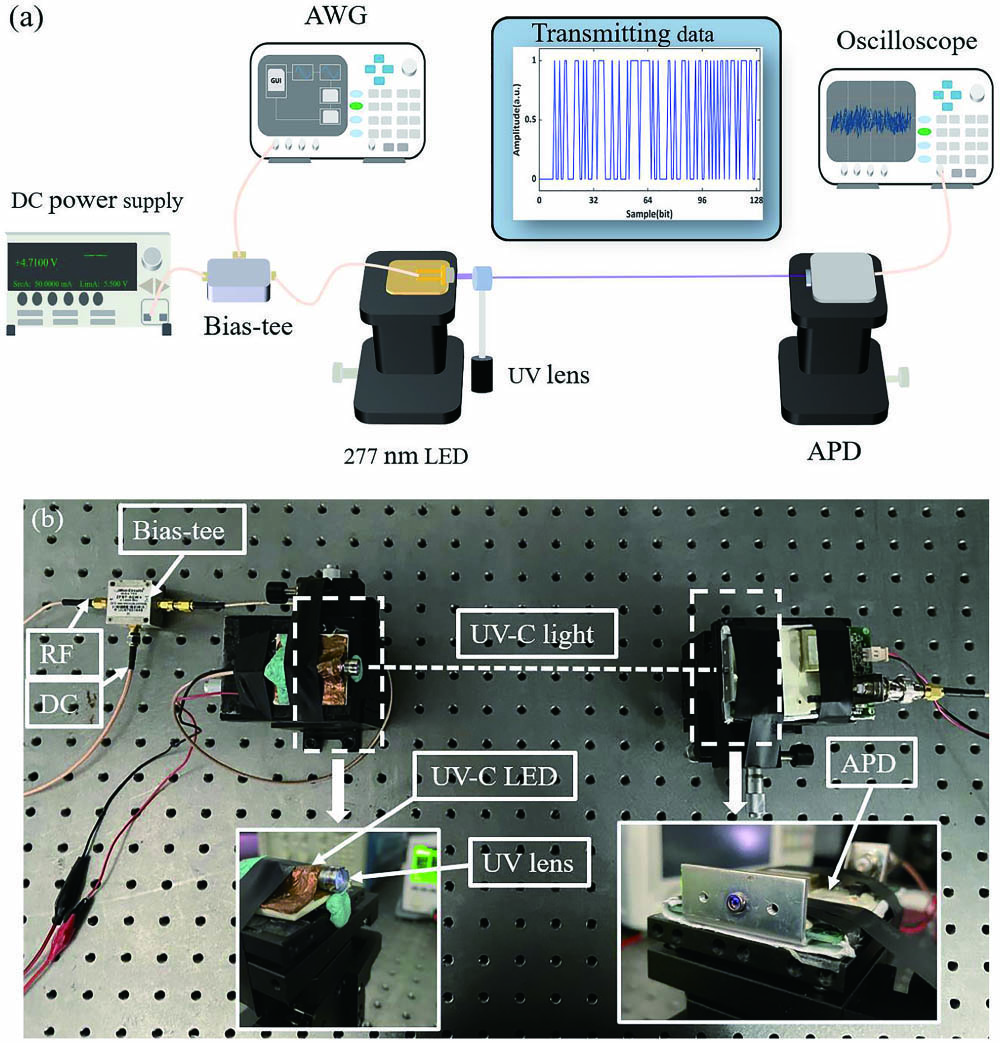
Set citation alerts for the article
Please enter your email address