Abstract
We present a compact Tm-doped fiber laser (TDFL) to generate pulse bursts at 1.92 μm based on phase and intensity modulations. A phase modulator (PM) and an intensity modulator (IM) were included in the linear TDFL cavity to perform the simultaneous active intracavity phase and intensity modulation. Stable pulse bursts have been achieved with tunable repetition rate in the range of 36–44 kHz (modulated by the PM) and duration of about 9.6 μs. The repetition rate of the individual pulse in a burst is about 9 MHz (modulated by the IM), and the pulse width is about 6 ns. By changing the IM signal’s repetition rate and duty cycle, different individual pulse shapes are obtained with pulse durations between 6 and 34 ns.1. INTRODUCTION
Pulse bursts are normally bundles of pulses at relatively low repetition rate and wide duration, composed of plentiful individual pulses with higher repetition rate and narrower pulse width [1–4]. Compared with conventional pulse trains, pulse bursts with clusters of subpulses have the advantages of ablating material at a faster speed while minimizing the thermal effects [3,4]. Moreover, pulse bursts almost enable the free handling of pulse trains to optimize the special work quality anticipated in a variety of applications [2–7], including material micromachining, laser acceleration, and environment measurements. Thus pulse burst fiber lasers have been widely investigated in recent years, mostly at the 1 μm band [8–15]. Kalaycioglu et al. reported the pulse bursts with 1 kHz repetition rate and 20 μJ individual pulse energy in 2011 [13], and they subsequently presented the 1 mJ pulse bursts in an Yb-doped fiber amplifier in 2012 [14]. Later in 2012, Breitkopf et al. pushed the energy power of pulse bursts at 1 μm to 58 mJ with burst repetition rates of 20 Hz [15]. The excellent investigations above actually make the 1 μm pulse bursts available for most of the current practical applications.
Different from Yb-doped fiber lasers, Tm-doped fiber lasers (TDFLs) have a broader emission band covering from to , which corresponds to various absorption bands of material, such as water and greenhouse gases [16,17]. The absorption at spectral lines of greenhouse gases enables TDFLs to be employed in environment monitoring and gas sensing [16,17]. Furthermore, high absorption by water makes TDFLs outstanding eye-safe laser sources in a variety of applications, including material micromachining, LIDAR, free-space communications, and medical procedures [17–19]. Thus TDFLs near 2 μm have attracted intense attention and have the potential to open a whole exotic area in laser industries [20,21]. Therefore pulse bursts generated by TDFLs are also in great demand and should be well investigated. Up to now, there have been no reports on pulse-burst generation in TDFLs, to the best of our knowledge.
The conventional method to generate pulse bursts is to tailor the pulse trains from an ultra-fast oscillator with an acousto-optic modulator (AOM), and the reported pulse bursts are mainly composed of picosecond or femtosecond individual pulses [13–15]. Actually, pulse bursts with nanosecond individual pulses and pulse spacing of tens of nanoseconds also have significant applications [22–26]. Furthermore, an individual pulse’s shape in the pulse bursts plays an important role when applying the pulse in practical applications [6,7]. For example, a step-flat top pulse in a material process has the advantage of initiating the process by a high-intensity pulse part and continuing the procedure with a lower intensity pulse part [27]. Actually, it has been demonstrated that simultaneous Q switching and mode locking can generate a burst-like pulse train from a fiber cavity with two AOMs based on intensity/amplitude modulations [28]. In addition, multiple pulses in gain-switched TDFLs have also been investigated [29,30]. But generation of bursts with shape-alterable pulses in TDFLs based on simultaneous active intracavity phase and intensity modulation has not been investigated yet.
Sign up for Photonics Research TOC. Get the latest issue of Photonics Research delivered right to you!Sign up now
In this paper, we propose and present a compact TDFL with monolithic configuration to generate pulse bursts with shape-alterable nanosecond individual pulses. A phase modulator (PM) and an intensity modulator (IM) are employed in the laser to perform the simultaneous active intracavity phase and intensity modulation. The repetition rate of the bursts can be tuned in the range of 36–44 kHz with a duration of about 9.6 μs, which is modulated by the PM. The repetition rate of the individual pulse is about 9 MHz with duration of 6 ns, which is determined by the cavity length and modulated by the IM. The individual pulse’s shape can be modified by adjusting the repetition rate and duty cycle of the signals on the IM. This is the first demonstration of 2 μm bursts with a shape-alterable pulse based on phase and intensity modulation, as far as we know.
2. EXPERIMENTAL SETUP
Figure 1 shows the experimental setup of the TDFL for pulse burst generation. A home-made 1570 nm fiber laser served as the pump laser, and the pump light was launched into the linear cavity through a high reflectivity fiber Bragg grating (HR FBG) with bandwidth of 1 nm and reflectivity of 99% at 1922.5 nm. A piece of 3 m long single cladding Tm-doped fiber with core diameter of 9 μm and cladding diameter of 125 μm was used as the gain medium. The core absorption efficiency of the Tm-doped fiber at 1570 nm was about . A PM and an IM were inserted in the cavity to perform active intracavity modulation. The electro-optical bandwidth of the phase modulator was about 100 MHz and that of the intensity modulator was about 10 GHz. The insertion losses of the IM and PM are about 0.5 and 0.6, respectively. The pulse bursts were launched out via an output coupler fiber Bragg grating (OC FBG) at 1922.5 nm with bandwidth of 0.5 nm and reflectivity of about 20%. The total length of the linear cavity was about 11.2 m. All the fiber components in the laser cavity were from commercial producers. The pulse train was monitored by a high-speed InGaAs PIN photodetector (PD) with bandwidth of 7 GHz and digital phosphor oscilloscope with bandwidth of 1 GHz. The spectrum of the laser was measured by an optical spectrum analyzer (OSA) with resolution of 0.05 nm.
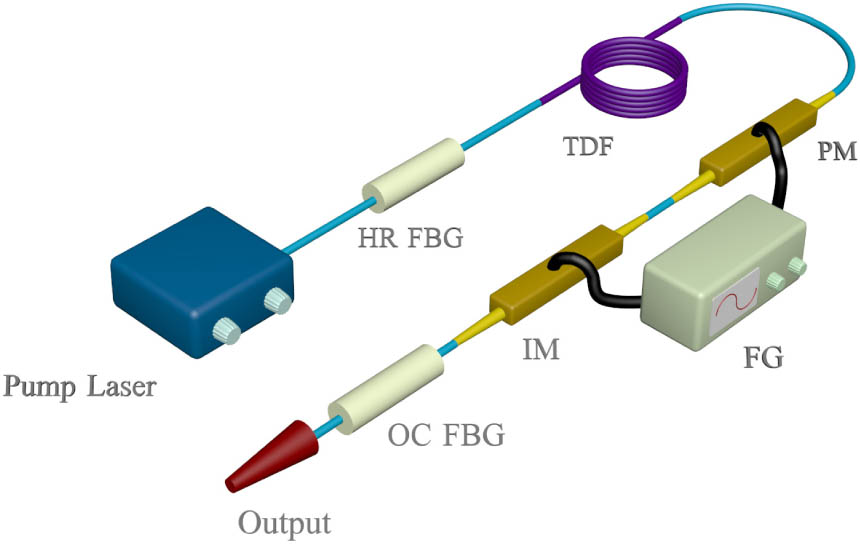
Figure 1.Experimental setup of the TDFL. TDF: Tm-doped fiber; PM: phase modulator; IM: intensity modulator; and FG: function generator.
3. EXPERIMENTAL RESULTS AND ANALYSIS
When both of the IM and PM signals were off, the TDFL operated in the continuous wave regime. The threshold of the laser was about 390 mW, and the pump power of the 1570 nm fiber laser was set to about 500 mW in the experiment. Pulse bursts can be generated when the two modulators were both working, as shown in Fig. 2. The modulation signal on the IM was a square pulse wave with repetition rate of 9 MHz and amplitude of 10 V; the duty cycle of the pulse wave was 50%. The modulation signal on the PM was a sinusoidal wave with frequency of 21.5 kHz and amplitude of 5 V. One can see from Fig. 2(a) that the bursts are orderly oscillating with dense subpulses, and the repetition rate of the pulse bursts is about 43 kHz. The duration of the pulse bursts is about 9.6 μs, and there are about 80 subpulses in one burst, as shown in Fig. 2(b). The subpulses’ repetition rate is 9 MHz, and the width is about 6 ns, as shown in Fig. 2(c). Moreover, the frequency of the signal on the PM can be tuned, and the repetition rate of the pulse bursts can also be altered along with that of the PM’s signal. The tuning range of the stable and uniform pulse bursts’ repetition rate covers from 36 to 44 kHz. Furthermore, the tuning range can be extended by changing the parameters of the laser cavity, such as the pump power, the length of the cavity, the material of the gain fiber, and the reflectivity of the OC FBG [31–35].
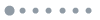
Figure 2.(a) Oscilloscope traces of pulse bursts train, (b) single pulse burst, and (c) individual pulses in one burst.
However, the pulse bursts presented somewhat unstable oscillation when the control signals were not well optimized. We attribute this relative unstability to the mode beating in the cavity. The IM in our experiment was electro-optical modulator (EOM)-based on an M-Z interferometer configuration, which inevitably resulted in two oscillating routes of the laser modes in the cavity with slightly different optical paths. Thus mode beating may appear, and turbulence of the pulses’ performance occurs. We believe that using an AOM instead of the EOM to perform the intensity modulation will intrinsically avoid the mode beating and can be employed to further improve the performance of the pulse bursts.
To analyze the generation process of pulse bursts, we turned off the phase modulation signal and performed the intensity modulation only, meanwhile monitoring the output pulse train, as shown in Fig. 3. One can find that the repetition rate of the continuous pulse train is 9 MHz, and the width of each pulse is about 6 ns, which is identical with those of the subpulses in pulse bursts as shown in Fig. 2(c). The duration of the adjacent pulse is measured to be about 110 ns, which approximately corresponds to the one-round-trip time of the pulse in the cavity. Using the equation , where is the estimated length of the cavity, we can obtain the mode-locking frequency of around 9.24 MHz. It is well known that only when the frequency of the IM’s signal is nearly identical with (or integer multiples of) the longitudinal mode frequency interval of the linear cavity can a stable pulse train with a higher optical signal-to-noise ratio be generated. In our experiment, the pulse train with the best performance was generated when the intensity modulation’s frequency was 9 MHz (or 18 MHz). So the actual mode-locking frequency should be around 9 MHz, and the difference between the calculated and actual mode-locking frequency mainly comes from the measure error of cavity length. Thus the control signals on the IM can generate the actively “mode-locked” pulse train based on intensity/amplitude modulation, and these pulses are exactly the subpulses in the pulse bursts.
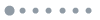
Figure 3.(a) Dense pulse train when only intensity modulation is on and (b) zoomed-in pulse train.
The effect of the PM in the pulse bursts’ generation can be investigated by turning off the intensity modulation signal and imposing the previous phase modulation only. The dynamic laser output monitored by the PD is shown in Fig. 4. It can be found that the laser is operating in the pulse regime. The frequency of phase modulation for Fig. 4 is about 21.5 kHz, which is indeed half the pulse frequency. The difference of periods in Fig. 4 can be attributed to the influence of the intensity modulator, which brings different optical paths in the cavity, although it is not working. However, the fluctuation of the period is mitigated well when the intensity modulator is working. Furthermore, the repetition rate of the pulse train is the same as that of the pulse bursts in Fig. 2(a). The generation of this kind of pulse by phase modulation has been previously investigated and related to phase modulation upon relaxation oscillation [31–35]. When the phase modulation frequency is above the minimum repetition frequency permitted [31,33], one can generate a pulse train typically at the modulation frequency or twice the modulation frequency when control parameters are optimized. In our experiment, when the phase-modulation signal was tuning by several kilohertz around 20 kHz or 40 kHz, a low-frequency pulse train with a repetition rate in the range of 36–44 kHz was obtained. Comparing the traces in Figs. 2(a), 3(a), and 4, it is not difficult to conclude that the phase modulation actually provided the Q-switching function and “tailored” the pulse bursts. However, this approach is not the same as methods for generation of bursts through directly cutting the high repetition rate pulse trains with IMs (AOM, for example) because it actually brings out the advantages of no direct energy loss. The signal-to-noise ratio (SNR) is about 32 dB when only the PM is on; the SNR is about 45 dB when both the PM and the IM are on; the SNR is about 55 dB when only the IM is on.
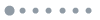
Figure 4.Pulse train when only phase modulation is on.
By slightly changing the repetition rate and duty cycle of the pulse control signals on the IM, we can alter the shape of the individual pulses in the bursts while the stability of the bursts is well maintained. As shown in Fig. 5(a), the pulse shape with a sharp top and a subsequent flat top was generated when the repetition rate of the control signal on IM was 9.41 MHz (slightly different from the mode-locking frequency of 9 MHz) and the duty-cycle was 42.5% the width of generated pulse was about 34 ns. Figure 5(c) shows the pulse shape with step-flat top when the repetition rate was 9.38 MHz and the duty-cycle was 47.7% and the pulse width was about 30 ns. The pulse shape with round top is shown in Fig. 5(b). The control signal’s repetition rate was 9.57 MHz and the duty cycle was 89%. The pulse width was about 12 ns. In Fig. 5(d), the shape of an actively “mode locked” pulse is depicted. The signal’s frequency was 9 MHz, and the duty cycle was 50%. The pulse’s width was measured to be about 6 ns. This kind of pulse-shape modification can be interpreted to mean simply that the intensity modulation of the IM shaped the pulse each time the pulse passed through the IM, and the final variety of output pulse shapes can be generated with different control signal repetition rates and duty cycles after numerous bounce trips [27,36]. This pulse train with an alterable shape in bursts will extraordinarily enhance its practical applications in material processing and environment monitoring.
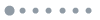
Figure 5.Oscilloscope traces of individual pulse shape control via intensity modulation. (a) Pulse shape with a sharp top and a subsequent flat top. (b) Pulse shape with round top. (c) Pulse shape with step-flat top. (d) Mode-locked pulse shape.
Figure 6 shows the spectra of the TDFL with pulse bursts in different cases. The central wavelength locates at 1922.4 nm when the laser is operating in the continuous wave regime. We can find that the spectra haven’t shown obvious degeneration in the other three cases, where only the PM’s signal is on, only the IM’s signal is on, and both signals are on. The full width at half-maximum (FWHM) of the laser’s spectrum is about 0.2 nm, which does not change much in all the operation cases in the experiment. Although the TDFL’s spectral linewidth and central wavelength vary a little, which may mainly be induced by the phase and intensity modulation on the laser modes, the spectrum’s rather favorable stability and uniformity can be ensured in this laser. Furthermore, by changing the wavelength of the FBGs in the laser cavity, laser sources of pulse bursts with other wavelengths near 2 μm are also available, which can be used in the wavelength-selective practical applications employing pulse bursts. The output average power is only about 5 mW and the bursts energy is about 0.125 μJ due to the relatively low power endurance of the fiber components (such as the IM and the PM). However, the higher output power of this pulse bursts laser can be achieved not only via more advanced devices that benefit from the prosperous development in commercial fiber components at 2 μm [20,21] but also by power amplification employing fiber amplifiers [14,15].
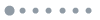
Figure 6.Spectra in different cases.
4. CONCLUSION
In conclusion, we present for the first time a compact TDFL at a 2 μm band for pulse bursts generation with shape-alterable subpulses. Intracavity active phase modulation and intensity modulation were employed simultaneously to generate the pulse bursts. The intensity modulation generated the active “mode locking” of the laser to form the individual pulses; meanwhile the phase modulation induced the active phase modulation on relaxation oscillation and hence generated the pulse bursts. The individual pulse’s shape in a burst can be modified by changing the repetition rate and duty cycle of the control signal on the IM. This kind of laser source provides a useful tool for various applications such as material processing, medicine care, and remote sensing employing 2 μm lasers. Further endeavors will be paid to improve the uniformity of the pulse bursts, variation of the pulse shape, and output energy.
References
[1] D. E. Leaird, A. M. Weiner, S. Kamei, M. Ishii, A. Sugita, K. Okamoto. Generation of flat-topped 500-GHz pulse bursts using loss engineered arrayed waveguide gratings. IEEE Photon. Technol. Lett., 14, 816-818(2002).
[2] R. R. Gattass, L. R. Cerami, E. Mazur. Micromachining of bulk glass with bursts of femtosecond laser pulses at variable repetition rates. Opt. Express, 14, 5279-5284(2006).
[3] X. Zeng, X. L. Mao, R. Greif, R. E. Russo. Experimental investigation of ablation efficiency and plasma expansion during femtosecond and nanosecond laser ablation of silicon. Appl. Phys. A, 80, 237-241(2005).
[4] J. König, S. Nolte, A. Tünnermann. Plasma evolution during metal ablation with ultrashort laser pulses. Opt. Express, 13, 10597-10607(2005).
[5] W. Hu, Y. C. Shin, G. King. Modeling of multi-burst mode pico-second laser ablation for improved material removal rate. Appl. Phys. A, 98, 407-415(2010).
[6] P. Deladurantaye, A. Cournoyer, M. Drolet, L. Desbiens, D. Lemieux, M. Briand, Y. Taillon. Material micromachining using bursts of high repetition rate picosecond pulses from a fiber laser. Proc. SPIE, 7914, 791404(2011).
[7] L. Desbiens, M. Drolet, V. Roy, M. M. Sisto, Y. Taillon. Arbitrarily-shaped bursts of picosecond pulses from a fiber laser source for high-throughput applications. Proc. SPIE, 7914, 791420(2011).
[8] T. S. McComb, D. McCal, R. Farrow, D. Logan, T. Lowder, C. Ye, T. Sosnowski, J. J. Koponen. Amplification of burst mode picosecond pulses to high peak powers in Chirally Coupled Core (3C) fibers. Advanced Solid-State Lasers Congress, JTh2A.36(2013).
[9] H. Kalaycioglu, B. Eldeniz, F. O. Ilday, K. Eken. Burst-mode Yb fiber amplifier producing 40 μJ individual pulse energy. CLEO: QELS-Fundamental Science, W2A-W81A(2012).
[10] R. Knappe. Applications of picosecond lasers and pulse-bursts in precision manufacturing. Proc. SPIE, 8243, 82430I(2012).
[11] A. Zadok, J. Sendowski, A. Yariv. Passively generated high repetition rate pulse bursts using a fiber laser with a polarization maintaining section. Conference on Lasers and Electro-Optics/International Quantum Electronics Conference(2009).
[12] V. García-Muñoz, M. A. Preciado, M. A. Muriel. Simultaneous ultrafast optical pulse train bursts generation and shaping based on Fourier series developments using superimposed fiber Bragg gratings. Opt. Express, 15, 10878-10889(2007).
[13] H. Kalaycıoğlu, K. Eken, F. Ö. Ilday. Fiber amplification of pulse bursts up to 20 μJ pulse energy at 1 kHz repetition rate. Opt. Lett., 36, 3383-3385(2011).
[14] H. Kalaycıoğlu, Y. B. Eldeniz, Ö. Akçaalan, S. Yavaş, K. Gürel, M. Efe, F. Ö. Ilday. 1 mJ pulse bursts from a Yb-doped fiber amplifier. Opt. Lett., 37, 2586-2588(2012).
[15] S. Breitkopf, A. Klenke, T. Gottschall, H. Otto, C. Jauregui, J. Limpert, A. Tünnermann. 58 mJ burst comprising ultrashort pulses with homogenous energy level from an Yb-doped fiber amplifier. Opt. Lett., 37, 5169-5171(2012).
[16] H. Sakata, S. Araki, T. Numano, M. Tomiki. All-fiber tunable Q-switched Tm fiber lasers for greenhouse gas sensing. Renewable Energy and the Environment Optics and Photonics Congress, ET4D.4(2012).
[17] J. Geng, Q. Wang, S. Jiang. 2 μm fiber laser sources and their applications. Proc. SPIE, 8164, 816409(2011).
[18] Z. Li, A. M. Heidt, N. Simakov, Y. Jung, J. M. O. Daniel, S. U. Alam, D. J. Richardson. Diode-pumped wideband thulium-doped fiber amplifiers for optical communications in the 1800–2050 nm window. Opt. Express, 21, 26450-26455(2013).
[19] J. Li, Z. Sun, H. Luo, Z. Yan, K. Zhou, Y. Liu, L. Zhang. Wide wavelength selectable all-fiber thulium doped fiber laser between 1925 nm and 2200 nm. Opt. Express, 22, 5387-5399(2014).
[20] . ISLA project aims to expand applications of 2-micron fiber laser technology(2011).
[21] . ISLA—Integrated disruptive components for 2 μm fibre lasers(2011).
[22] J. R. Salcedo. Dynamic pulsing of a MOPA pulsed fiber laser for optimized material processing. Lasers, Sources, and Related Photonic Devices, h2A-h31A(2012).
[23] S. T. Hendow, R. Romero, S. A. Shakir, P. T. Guerreiro. Percussion drilling of metals using bursts of nanosecond pulses. Opt. Express, 19, 10221-10231(2011).
[24] S. T. Hendow, S. A. Shakir. Structuring materials with nanosecond laser pulses. Opt. Express, 18, 10188-10199(2010).
[25] H. Herfurth, R. Patwa, T. Lauterborn, S. Heinemann, H. Pantsar. Micromachining with tailored nanosecond pulses. Proc. SPIE, 6796, 67961G(2007).
[26] P. Deladurantaye, D. Gay, A. Cournoyer, V. Roy, B. Labranche, B. M. Levesque, Y. Taillon. Material micromachining using a pulsed fiber laser platform with fine temporal nanosecond pulse shaping capability. Proc. SPIE, 7195, 71951S(2009).
[27] A. Malinowski, K. T. Vu, K. K. Chen, J. Nilsson, Y. Jeong, S. Alam, D. Lin, D. J. Richardson. High power pulsed fiber MOPA system incorporating electro-optic modulator based adaptive pulse shaping. Opt. Express, 17, 20927-20937(2009).
[28] M. Eckerle, C. Kieleck, J. Świderski, S. D. Jackson, G. Mazé, M. Eichhorn. Actively Q-switched and mode-locked Tm3+-doped silicate 2 μm fiber laser for supercontinuum generation in fluoride fiber. Opt. Lett., 37, 512-514(2012).
[29] B. C. Dickinson, S. D. Jackson, T. A. King. 10 mJ total output from a gain-switched Tm-doped fibre laser. Opt. Commun., 182, 199-203(2000).
[30] J. Yang, Y. Tang, J. Xu. Development and applications of gain-switched fiber lasers [Invited]. Photon. Res., 1, 52-57(2013).
[31] L. A. Zenteno, H. Po. Frequency-modulated cavity-dumped Nd-doped fiber laser. Opt. Lett., 16, 315-317(1991).
[32] R. Roy, K. S. Thornburg. Experimental synchronization of chaotic lasers. Phys. Rev. Lett., 72, 2009-2012(1994).
[33] D. Ostling, P. G. Sinha, H. E. Engan. Spectral stability and smoothness of a phase-modulated fiber laser. Opt. Lett., 20, 219-221(1995).
[34] S. Longhi, P. Laporta. Floquet theory of intracavity laser frequency modulation. Phys. Rev. A, 60, 4016-4028(1999).
[35] X. Wang, P. Zhou, X. Wang, R. Tao, L. Si. 2 μm Tm-doped all-fiber pulse laser with active mode-locking and relaxation oscillation modulating. IEEE Photon. J., 5, 1502206(2013).
[36] L. Escalante-Zarate, Y. O. Barmenkov, J. L. Cruz, M. V. Andrés. Q-switch modulator as a pulse shaper in Q-switched fiber lasers. IEEE Photon. Technol. Lett., 24, 312-314(2012).