
- Journal of Inorganic Materials
- Vol. 36, Issue 11, 1217 (2021)
Abstract
In the past few years, metal-halide perovskite quantum dots (QDs) have attracted enormous attention for its remarkable optoelectronic properties, such as excellent absorption ability, high-quantum-yield photoluminescence, tunable band gap, long carrier lifetime and so on[
Recently, two-dimensional (2D) materials have aroused extensive attention from researchers due to their exceptional electronic and optical properties compared with their bulk counterparts[
In this contribution, ultrathin g-C3N4 (UCN) nanosheets were employed to combine with CsPbBr3 QDs, with the aim of coupling 2D ultrathin g-C3N4 nanosheets with 0D CsPbBr3 QDs to obtain a 0D/2D composite photocatalyst with high performance. The photocatalytic performance of the obtained 0D/2D CsPbBr3/UCN composite was evaluated by the degradation of a typical pollutant in water, Rhodamine B (RhB). Benefitting from the suitable energy band structure, such a 0D/2D heterostructure exhibited enhanced photocatalytic activity than both pristine CsPbBr3 QDs and UCN. Furthermore, due to the strong interaction between CsPbBr3 QDs and UCN, the stability of CsPbBr3 QDs was greatly enhanced.
1 Experimental
1.1 Material preparation
1.1.1 Preparation of ultrathin g-C3N4 nanosheets
Bulk g-C3N4 was first prepared by pyrolysis of melamine at 550 ℃ for 4 h with a ramp rate of 2.5 ℃/min, which was donated as BCN. Ultrathin g-C3N4 nanosheets were synthesized by exfoliating BCN as follows: 320 mg of BCN was mixed with 6 mol/L HCl solution (80 mL), which was then transferred into a 100 mL Teflonlined autoclave and heated at 110 ℃ for 5 h. Upon cooling, the precipitate was filtered, washed and dried at 80 ℃ under vacuum for 12 h. The obtained ultrathin g-C3N4 nanosheets was donated as UCN.
1.1.2 Preparation of CsPbBr3 QDs
CsPbBr3 QDs was synthesized using a typical hot- injection method published by Protesescu et al[
1.1.3 Preparation of CsPbBr3/UCN composite
The preparation process of the CsPbBr3/UCN composite was illustrated in Scheme 1: 10 mg of the obtained CsPbBr3 QDs was dispersed in 10 mL toluene, then 90 mg of the as-prepared UCN was added into the CsPbBr3 QDs ink solution. After stirring for several hours, the color of ink solution turned from yellow to colorless, indicating that CsPbBr3 QDs have anchored on UCN. The precipitate was centrifuged to obtain the CsPbBr3/UCN composite.
Figure Scheme 1.Illustration of the preparation process of CsPbBr3/ UCN composite.
1.2 Characterization
The phase identification of the as-prepared products were characterized by X-ray diffraction (XRD, D/max 2200PC) using Cu Kα radiation. The morphologies of the products were observed by transmission electron microscope (TEM, FEI tecnaiG2F30) operated at 200 kV. The optical absorption spectra of the products were obtained from a PE Lambda 900 UV-visible spectrophotometer. The carrier separation ability of the samples were characterized by Photoluminescence (PL) spectra on a fluorescence spectrometer (FluoroMax-4) and photo-electrochemical experiments on an electrochemical system (CHI-650E) with three-electrode system.
1.3 Photocatalytic experiments
Photocatalytic experiments were carried out under a 500 W Xe lamp with 420 nm UV filter. In a typical procedure, 50 mg of as-prepared photocatalyst and 50 mL of RhB solution (10-5 mol/L) were mixed in a beaker. After being stirred in the dark for 60 min, the mixture was illuminated under visible light and 3 mL of the suspension was withdrawn at given intervals. After the photocatalyst particles being removed by centrifugation, the absorption spectral change of the supernatant was measured with a PE Lambda 900 UV-Vis spectrophotometer. The concentration change of RhB was obtained by monitoring the absorption band maximum at 552 nm.
2 Results and discussion
2.1 Crystal structure
The crystal structures of CsPbBr3 QDs, UCN and CsPbBr3/UCN composite were characterized by XRD patterns. As exhibited in Fig. 1, the peaks of the as-prepared CsPbBr3 QDs matched well with the orthorhombic CsPbBr3 (JCPDS 18-0364). For the UCN sample, two diffraction peaks at 2θ=13.2° and 27.7° can be observed, corresponding to the (100) and (002) diffraction planes of g-C3N4, respectively[
Figure 1.XRD patterns of CsPbBr3, UCN and CsPbBr3/UCN composite
2.2 Morphology analysis
TEM was employed to investigate the morphology of the as-fabricated CsPbBr3 QDs, UCN and CsPbBr3/UCN composite. Fig. 2(A) shows the TEM image of CsPbBr3 QDs, demonstrating a cubic-shaped morphology with fairy uniform size of around 10 nm. As shown in Fig. 2(B), the UCN sample is composed of thin nanosheets, indicating the successful exfoliation of bulk g-C3N4. In the TEM image of CsPbBr3/UCN composite (Fig. 2(C)), it can be seen that CsPbBr3 QDs are dispersed on UCN nanosheets. Moreover, a clear lattice spacing of 0.58 nm is observed from the HRTEM image of the CsPbBr3 QDs on UCN (Fig. 2(D)), which is attributed to the (100) plane of orthorhombic CsPbBr3[
Figure 2.TEM image of (A) CsPbBr3 QDs, (B) UCN and (C) CsPbBr3/UCN composite; (D) HRTEM image of CsPbBr3/ UCN composite
2.3 Optical properties
The optical properties of CsPbBr3 QDs, UCN and CsPbBr3/UCN composite were investigated by UV-Vis diffused absorption spectra, which were exhibited in Fig. 3. UCN displayed a visible absorption edge at 473 nm, which corresponded to a bandgap energy of 2.62 eV. CsPbBr3 QDs showed the absorption edge at around 550 nm, illustration of a bandgap energy of 2.25 eV. Compared to the pure CsPbBr3 QDs and pure UCN, a notable red-shift of the absorbance and enhanced light- harvesting of the CsPbBr3/UCN composite is observed, which can be ascribed to the synergistic effect of CsPbBr3 QDs and UCN, implying a more efficient utilization of visible light during the photocatalytic reaction.
Figure 3.UV-Vis diffuse reflectance spectra of CsPbBr3, UCN and CsPbBr3/UCN composite
2.4 Evalution of photocatalytic activity
In order to assess the practicability of the CsPbBr3/UCN composite in the photocatalytic applications, the degradation of RhB was performed. As shown in Fig. 4(A), the CsPbBr3/UCN composite exhibited noticeably improved photocatalytic activity than pure CsPbBr3 QDs and pure UCN, which can almost completely degrade RhB after 15 min visible light irradiation, accompanied with a total organic carbon (TOC) removal efficiency of 82.6%. The high degree of mineralization of organic species confirms that RhB has been photocatalytically decomposed. Moreover, to assess the superiority of UCN, the photocatalytic activity of bulk g-C3N4 (BCN) was also evaluated, which was much lower than that of UCN and CsPbBr3/UCN composite. In addition, the stability of the photocatalyst is an important factor limiting its practical application. As is known, CsPbBr3 QDs have poor water stability, since they are subjected to structural decomposition in aqueous phase[
Figure 4.(A) Photocatalytic degradation of RhB over CsPbBr3, BCN, UCN and CsPbBr3/UCN composite; (B) XRD patterns of recycled CsPbBr3 and CsPbBr3/UCN composite
As shown in Fig. 4(B), severe structure degradation is observed for the CsPbBr3 QDs after water immersion, while the crystal structure of the CsPbBr3/UCN composite is well preserved, demonstrating the good water stability of the CsPbBr3/UCN composite. The stability enhancement could be ascribed to the formation of N-Br bonding between CsPbBr3 QDs and UCN. Since amino group can interact strongly with CsPbBr3 QDs through the surface bromide, the resultant CsPbBr3/UCN composite is more stable than organic ligands passivation.
2.5 Photocatalytic mechanism
For an ideal photocatalyst, the expanded spectral response scope, and the effective charge separation and transportation ability are desired. As shown in Fig. 3, the spectral response scope of the CsPbBr3/UCN composite were obviously extended compared with pure CsPbBr3 and UCN, indicating more light-harvesting of the CsPbBr3/UCN composite. In order to investigate the photon-generated charge separation and migration abilities of the as-prepared products, PL spectra and transient photocurrent response experiments were performed. As shown in Fig. 5(A), CsPbBr3 QDs exhibited a strong emission peak at 520 nm, while anchoring CsPbBr3 on the UCN drastically reduced the PL intensity. Besides, the emission intensity originated from UCN also decreased slightly, implying the occurrence of photo-generated carrier transfer between CsPbBr3 and UCN. Moreover, the transient photocurrent response spectra (Fig. 5(B)) showed that the CsPbBr3/UCN composite exhibited significantly higher photocurrent response intensities than both CsPbBr3 QDs and UCN, which indicated more efficient charge transfer of the CsPbBr3/UCN composite.
Figure 5.(A) PL spectra and (B) transient photocurrent responses of CsPbBr3, UCN and CsPbBr3/UCN composite
Mott-Schottky measurements were performed to determine the band potentials of UCN and CsPbBr3. As shown in Fig. 6(A), both UCN and CsPbBr3 feature a typical n-type semiconductor, with the flat band potential values of -1.01 and -0.91 eV versus the saturated calomel electrode (SCE), respectively. Correspondingly, the valance band positions for UCN and CsPbBr3 are 1.61 and 1.14 eV (vs. NHE) based on the calculated bandgap, respectively. Hence, the band alignments of both samples are illustrated in Fig. 6(B). It is clear that the conduction band minimum (CBM) of UCN is lower than that of CsPbBr3, and the valence band maximum (VBM) of UCN is lower than that of CsPbBr3. Therefore, the conduction band electrons of CsPbBr3 can transfer to that of UCN, while the valence band holes of UCN can migrate to that of CsPbBr3. As such, effective charge separation and inhibition of charge recombination in the composite photocatalyst is realized, which led to the improved photocatalytic activity.
Figure 6.(A) Electrochemical Mott-Schottky plots and (B) band alignments of CsPbBr3 and UCN
3 Conclusions
In summary, we have successfully fabricated a CsPbBr3/UCN composite via a facile process, which can be used as an efficient and stable photocatalyst in water medium. Microstructure characterization showed that the CsPbBr3 QDs were anchored on the surface of UCN to form a 0D/2D heterostructure. The formation of the CsPbBr3/UCN heterostructure can greatly facilitate photo-generated charge separation and transportation, as disclosed by the PL spectra and the photoelectrochemical measurements. The expanded light-harvesting scope, as well as the enhanced charge separation efficiency are responsible for the improved photocatalytic activity of the CsPbBr3/UCN composite. This work may provide a useful guide towards the utilization of metal halide perovskite for photocatalytic applications.
References
[15] M OU, G TU W, G YIN S et al. Amino-assisted anchoring of CsPbBr3 perovskite quantum dots on porous g-C3N4 for enhanced photocatalytic CO2 reduction. Angewandte Chemie International Edition, 130, 13758-13762(2018).
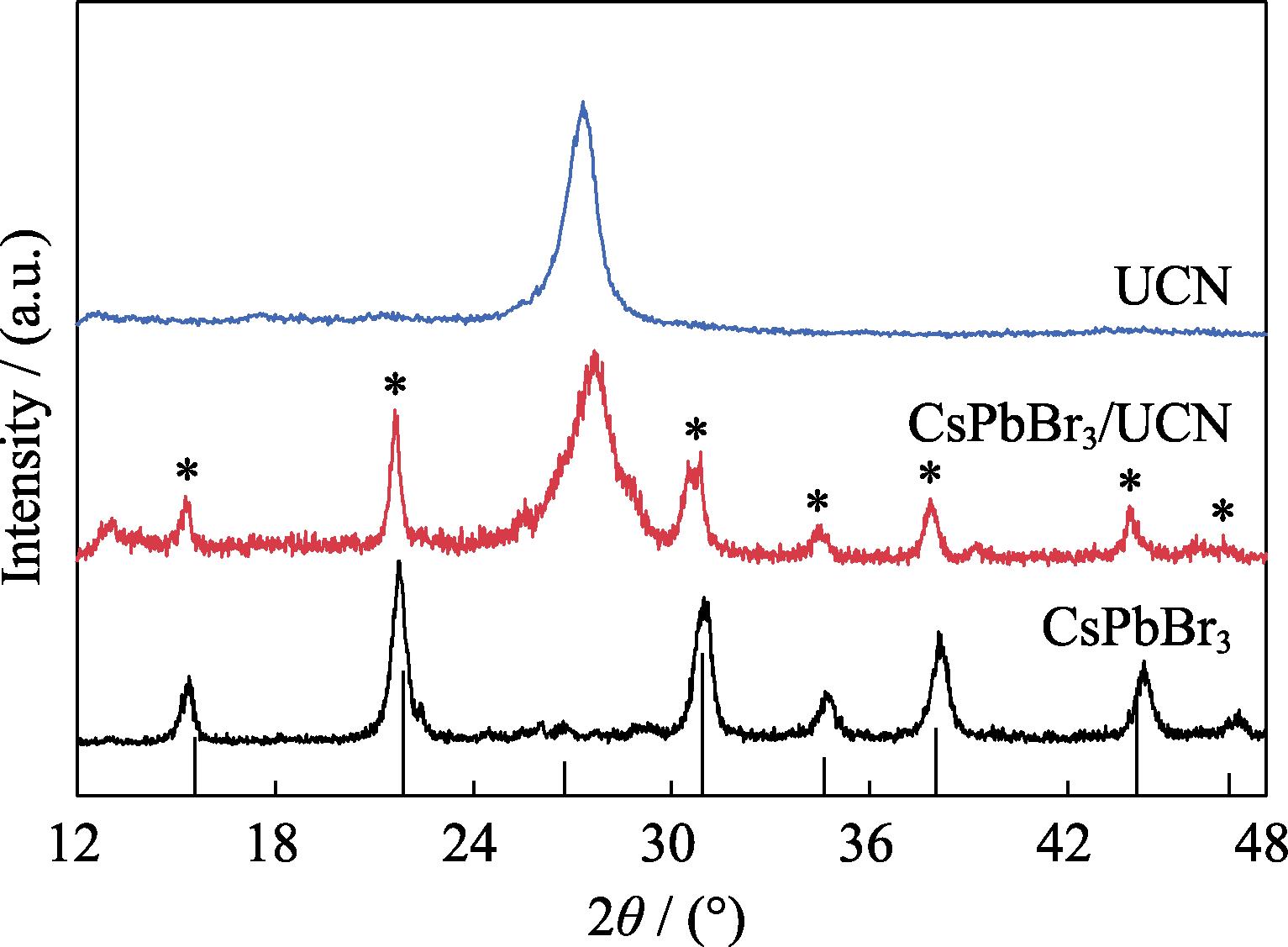
Set citation alerts for the article
Please enter your email address