
- Photonics Research
- Vol. 10, Issue 9, 2008 (2022)
Abstract
1. INTRODUCTION
Metamaterials can flexibly control the amplitude, phase, and polarization of light at the sub-wavelength scale. Their appearance has mostly changed the way in which we manipulate electromagnetic waves, thus breaking through many limitations of traditional optics. Due to their unique and novel properties, metamaterials play an important role in various fields and realize a variety of functions, such as holographic imaging [1–3], beam deflectors [4–6], perfect absorbers [7–10], and flat lenses [11–15]. With the expansion of application fields, higher requirements are put forward for metamaterials, especially for functionally decoupling metamaterials that can integrate different functions into a single device. At present, the common method of decoupling functions of metamaterials is spin decoupling with circular polarization incidence [16–21], which realizes polarization-related components by simultaneously controlling the phase of orthogonally polarized waves, thereby realizing different functions. However, this method cannot realize flexibly dynamic function switching because it needs to switch the polarization state of the electromagnetic signal to realize different functions. It is evident that a dynamic metamaterial with response to external excitation signals is a potential approach.
At microwave frequencies, electronically controlled metasurfaces [22–26] with integrated diodes have become a very attractive control method due to their tuning flexibility. However, when this method is extended to higher frequencies, there is a lack of corresponding functional electronic devices, and it is quite difficult to integrate electrodes into each structure. Therefore, in the terahertz (THz) band, many researchers are committed to realizing actively tunable metamaterials by introducing materials with external field induction response, such as two-dimensional material graphene [27–30], liquid crystal [31–34], and phase change materials [3,35]. Especially, vanadium dioxide (
In this paper, we experimentally demonstrate a dynamic bifunctional metamaterial embedded with
Sign up for Photonics Research TOC. Get the latest issue of Photonics Research delivered right to you!Sign up now
As a verification, we experimentally demonstrate a thermally-controlled tunable THz off-axis parabolic mirror embedded with
Figure 1.Schematic diagram of dynamic function switching of THz waves via mode decoupling controlled by external physical field stimulus. When
2. DESIGN AND THEORY
As a phase change material, the resistivity of
For the design in this paper, the composite superatom structure of metal combined with
Figure 2.(a) Schematic diagram of the unit structure and parameter annotation. (b) Equivalent structure diagram, (c) surface current distribution, and (d) corresponding S-mode field distribution of the unit in the insulating state at normal temperature. (e) Equivalent structure diagram, (f) surface current distribution, and (g) corresponding D-mode field distribution of the unit in the metallic state at high temperature.
Due to the near-field interaction between the metal layers, the superatom supports the typical gap–surface plasmon resonance, forming a gap–surface plasmon metasurface and realizing orthogonal polarization transformation. In this way, each superatom can simultaneously and independently manipulate the polarization and phase of the reflected field at a thickness of sub-wavelength scale. At room temperature of 300 K, as shown in Fig. 2(b),
Here, by introducing the Jones matrix [48], the relationship between reflected wave and incident wave can be expressed as follows [30,49]:
More importantly, we separately scanned the phase delay and reflectivity of the cross-polarized wave in the
Figure 3.(a) Amplitude of reflected cross-polarization wave in S-mode at room temperature and (b) corresponding phase delay. (c) Amplitude of reflected cross-polarization wave in high-temperature D-mode and (d) corresponding phase delay.
Since the amplitudes of the last four elements are equal to the first four and the phase difference in the working frequency band is 180°, their reflection spectrum curves are no longer listed separately, and only their amplitude and phase characteristics are given at the 340 GHz frequency point. It can be seen from Figs. 4(a) and 4(b) that the cross-polarization phases of the selected units in both modes are linearly distributed with a higher amplitude, and the gradient is uniform in the working band. Further, we extracted the amplitude and phase characteristics of the selected unit at the atmospheric window frequency point of 340 GHz. As shown in Figs. 4(c) and 4(d), the cross-polarized wave amplitude in the S-mode is higher with certain fluctuations, while in the D-mode, the amplitude of the cross-polarized wave is relatively low, but the fluctuations are gentle and the consistency is better. The selected units in both modes have a phase gradient of 45° and can achieve 360° phase coverage under polarization conversion. According to the theory of coding metamaterials [51], we correspond to the phase “0°, 45°, 90°, 135°, 180°, 225°, 270°, 315°” of the selected cells in the two modes with “000, 001, 010, 011, 100, 101, 110, 111,” respectively, so that the independent 3-bit coding design can be carried out in the two modes.
Figure 4.(a) Spectra of the reflected cross-polarized wave (top) and corresponding phase delay (bottom) for forward illuminated
3. RESULTS AND DISCUSSION
To test the performance of the tunable THz metasurface we propose, we designed a zoomable off-axis parabolic mirror function for verification. According to the following Eq. (4) [52], we can calculate the phase distribution required for different focal positions:
According to the corresponding phase encoding distribution, we arrange the superatom array and simulate the working state of the array in both modes. At room temperature of 300 K,
Figure 5.Simulation and experimental results of the reflected cross-polarized wave focusing off-axis with tunable focal length when
When the temperature of the active area increases above 341 K, the conductivity of
Since the structure we propose is completely independent in the two modes, it can achieve not only phase compensation but also phase reconstruction, ensuring the functional decoupling of THz wave modulation in the two modes. Thereby, the metasurface can change not only the focal length but also the focus direction at a large angle by changing the coding combination of the two modes. At room temperature of 300 K,
Figure 6.Simulation and experimental results of the reflected cross-polarized wave focusing off-axis with large-angle focus deflection when
When the designed metasurface is physically heated,
By comparison, we find that the effective conversion efficiency of the cross-polarized wave of the experimental results is lower than that of the simulation results. This is likely due to the conductivity and permittivity of
4. EXPERIMENT
The processing of the
Figure 7.(a) Image of a fabricated metasurface sample and (b) its partially enlarged view. (c) Schematic diagram of the experimental setup.
The measuring device is shown in Fig. 7(c). The 0.34 THz
5. CONCLUSION
In this paper, we propose a dynamic bifunctional metasurface that combines patterned nano-scale
Acknowledgment
Acknowledgment. Thanks to Sichuan University, Hebei Semiconductor Research Institute, and Suzhou Institute of Nano-Tech and Nano-Bionics (SINANO) for their help with this work.
References
[1] D. Wen, F. Yue, G. Li, G. Zheng, K. Chan, S. Chen, M. Chen, K. F. Li, P. W. H. Wong, K. W. Cheah, E. Y. B. Pun, S. Zhang, X. Chen. Helicity multiplexed broadband metasurface holograms. Nat. Commun., 6, 8241(2015).
[2] L. Jin, Z. Dong, S. Mei, Y. F. Yu, Z. Wei, Z. Pan, S. D. Rezaei, X. Li, A. I. Kuznetsov, Y. S. Kivshar, J. K. W. Yang, C.-W. Qiu. Noninterleaved metasurface for (26–1) spin- and wavelength-encoded holograms. Nano Lett., 18, 8016-8024(2018).
[3] X. Liu, Q. Wang, X. Zhang, H. Li, Q. Xu, Y. Xu, X. Chen, S. Li, M. Liu, Z. Tian, C. Zhang, C. Zou, J. Han, W. Zhang. Thermally dependent dynamic meta-holography using a vanadium dioxide integrated metasurface. Adv. Opt. Mater., 7, 1900175(2019).
[4] Y. Yuan, K. Zhang, X. Ding, B. Ratni, S. N. Burokur, Q. Wu. Complementary transmissive ultra-thin meta-deflectors for broadband polarization-independent refractions in the microwave region. Photon. Res., 7, 80-88(2019).
[5] X. Yin, H. Zhu, H. Guo, M. Deng, T. Xu, Z. Gong, X. Li, Z. H. Hang, C. Wu, H. Li, S. Chen, L. Zhou, L. Chen. Hyperbolic metamaterial devices for wavefront manipulation. Laser Photon. Rev., 13, 1800081(2019).
[6] L. Zhang, J. Ding, H. Zheng, S. An, H. Lin, B. Zheng, Q. Du, G. Yin, J. Michon, Y. Zhang, Z. Fang, M. Y. Shalaginov, L. Deng, T. Gu, H. Zhang, J. Hu. Ultra-thin high-efficiency mid-infrared transmissive Huygens meta-optics. Nat. Commun., 9, 1481(2018).
[7] W. Wang, L. V. Besteiro, T. Liu, C. Wu, J. Sun, P. Yu, L. Chang, Z. Wang, A. O. Govorov. Generation of hot electrons with chiral metamaterial perfect absorbers: giant optical chirality for polarization-sensitive photochemistry. ACS Photon., 6, 3241-3252(2019).
[8] A. Ghobadi, H. Hajian, B. Butun, E. Ozbay. Strong light–matter interaction in lithography-free planar metamaterial perfect absorbers. ACS Photon., 5, 4203-4221(2018).
[9] B. Tang, Z. Li, E. Palacios, Z. Liu, S. Butun, K. Aydin. Chiral-selective plasmonic metasurface absorbers operating at visible frequencies. IEEE Photon. Technol. Lett., 29, 295-298(2017).
[10] Y. Ren, T. Zhou, C. Jiang, B. Tang. Thermally switching between perfect absorber and asymmetric transmission in vanadium dioxide-assisted metamaterials. Opt. Express, 29, 7666-7679(2021).
[11] Q. Wang, X. Zhang, Y. Xu, Z. Tian, J. Gu, W. Yue, S. Zhang, J. Han, W. Zhang. A broadband metasurface-based terahertz flat-lens array. Adv. Opt. Mater., 3, 779-785(2015).
[12] Z. Wang, T. Yang, Y. Zhang, Q. Ou, H. Lin, Q. Zhang, H. Chen, H. Y. Hoh, B. Jia, Q. Bao. Flat lenses based on 2D perovskite nanosheets. Adv. Mater., 32, 2001388(2020).
[13] X.-T. Kong, A. A. Khan, P. R. Kidambi, S. Deng, A. K. Yetisen, B. Dlubak, P. Hiralal, Y. Montelongo, J. Bowen, S. Xavier, K. Jiang, G. A. J. Amaratunga, S. Hofmann, T. D. Wilkinson, Q. Dai, H. Butt. Graphene-based ultrathin flat lenses. ACS Photon., 2, 200-207(2015).
[14] F. Ding, Y. Chen, S. I. Bozhevolnyi. Gap-surface plasmon metasurfaces for linear-polarization conversion, focusing, and beam splitting. Photon. Res., 8, 707-714(2020).
[15] W. Ma, D. Jia, X. Yu, Y. Feng, Y. Zhao. Reflective gradient metasurfaces for polarization-independent light focusing at normal or oblique incidence. Appl. Phys. Lett., 108, 071111(2016).
[16] C. Zheng, J. Li, G. Wang, J. Li, S. Wang, M. Li, H. Zhao, Z. Yue, Y. Zhang, Y. Zhang, J. Yao. All-dielectric chiral coding metasurface based on spin-decoupling in terahertz band. Nanophotonics, 10, 1347-1355(2021).
[17] A. Arbabi, Y. Horie, M. Bagheri, A. Faraon. Dielectric metasurfaces for complete control of phase and polarization with subwavelength spatial resolution and high transmission. Nat. Nanotechnol., 10, 937-943(2015).
[18] J. P. Balthasar Mueller, N. A. Rubin, R. C. Devlin, B. Groever, F. Capasso. Metasurface polarization optics: independent phase control of arbitrary orthogonal states of polarization. Phys. Rev. Lett., 118, 113901(2017).
[19] B. Yao, X. Zang, Y. Zhu, D. Yu, J. Xie, L. Chen, S. Han, Y. Zhu, S. Zhuang. Spin-decoupled metalens with intensity-tunable multiple focal points. Photon. Res., 9, 1019-1032(2021).
[20] G. Ding, K. Chen, X. Luo, J. Zhao, T. Jiang, Y. Feng. Dual-helicity decoupled coding metasurface for independent spin-to-orbital angular momentum conversion. Phys. Rev. Appl., 11, 044043(2019).
[21] Y. Xu, Q. Li, X. Zhang, M. Wei, Q. Xu, Q. Wang, H. Zhang, W. Zhang, C. Hu, Z. Zhang, C. Zhang, X. Zhang, J. Han, W. Zhang. Spin-decoupled multifunctional metasurface for asymmetric polarization generation. ACS Photon., 6, 2933-2941(2019).
[22] L. Chen, Q. Ma, Q. F. Nie, Q. R. Hong, H. Y. Cui, Y. Ruan, T. J. Cui. Dual-polarization programmable metasurface modulator for near-field information encoding and transmission. Photon. Res., 9, 116-124(2021).
[23] S. J. Li, Y. B. Li, L. Zhang, Z. J. Luo, B. W. Han, R. Q. Li, X. Y. Cao, Q. Cheng, T. J. Cui. Programmable controls to scattering properties of a radiation array. Laser Photon. Rev., 15, 2000449(2021).
[24] W. Tang, J. Y. Dai, M. Chen, X. Li, Q. Cheng, S. Jin, K. Wong, T. J. Cui. Programmable metasurface-based RF chain-free 8PSK wireless transmitter. Electron. Lett., 55, 417-420(2019).
[25] J. Zhao, X. Yang, J. Y. Dai, Q. Cheng, X. Li, N. H. Qi, J. C. Ke, G. D. Bai, S. Liu, S. Jin, A. Alù, T. J. Cui. Programmable time-domain digital-coding metasurface for non-linear harmonic manipulation and new wireless communication systems. Natl. Sci. Rev., 6, 231-238(2019).
[26] W. Tang, M. Z. Chen, X. Chen, J. Y. Dai, Y. Han, M. Di Renzo, Y. Zeng, S. Jin, Q. Cheng, T. J. Cui. Wireless communications with reconfigurable intelligent surface: path loss modeling and experimental measurement. IEEE Trans. Wirel. Commun., 20, 421-439(2021).
[27] P. Ding, Y. Li, L. Shao, X. Tian, J. Wang, C. Fan. Graphene aperture-based metalens for dynamic focusing of terahertz waves. Opt. Express, 26, 28038-28050(2018).
[28] J. Huang, B. Hu, K. Muhammad Ismail, W. Liu, J. Liu. Graphene-enabled active terahertz focusing with wide tuning range. J. Phys. D, 54, 385104(2021).
[29] W. Liu, B. Hu, Z. Huang, H. Guan, H. Li, X. Wang, Y. Zhang, H. Yin, X. Xiong, J. Liu, Y. Wang. Graphene-enabled electrically controlled terahertz meta-lens. Photon. Res., 6, 703-708(2018).
[30] B. Tang, Y. Ren. Tunable and switchable multi-functional terahertz metamaterials based on a hybrid vanadium dioxide–graphene integrated configuration. Phys. Chem. Chem. Phys., 24, 8408-8414(2022).
[31] S. Zhou, Z. Shen, X. Li, S. Ge, Y. Lu, W. Hu. Liquid crystal integrated metalens with dynamic focusing property. Opt. Lett., 45, 4324-4327(2020).
[32] Z. Shen, S. Zhou, X. Li, S. Ge, P. Chen, W. Hu, Y. Lu. Liquid crystal integrated metalens with tunable chromatic aberration. Adv. Photon., 2, 036002(2020).
[33] Z. Shen, S. Zhou, S. Ge, W. Duan, L. Ma, Y. Lu, W. Hu. Liquid crystal tunable terahertz lens with spin-selected focusing property. Opt. Express, 27, 8800-8807(2019).
[34] Z. Shen, M. Tang, P. Chen, S. Zhou, S. Ge, W. Duan, T. Wei, X. Liang, W. Hu, Y. Lu. Planar terahertz photonics mediated by liquid crystal polymers. Adv. Opt. Mater., 8, 1902124(2020).
[35] C. Zhang, G. Zhou, J. Wu, Y. Tang, Q. Wen, S. Li, J. Han, B. Jin, J. Chen, P. Wu. Active control of terahertz waves using vanadium-dioxide-embedded metamaterials. Phys. Rev. Appl., 11, 054016(2019).
[36] B. K. Shrewsbury, A. M. Morsy, M. L. Povinelli. Multilayer planar structure for optimized passive thermal homeostasis [Invited]. Opt. Mater. Express, 12, 1442-1449(2022).
[37] J.-L. Fang, L. Qu, H.-L. Yi. Thermal switching of near-field radiative heat transfer between nanoparticles via multilayered surface modes. Phys. Rev. Appl., 17, 034040(2022).
[38] K. Nishikawa, M. Yoshimura, Y. Watanabe. Growth of nanostructured VO2 via controlling oxidation of V thin films: morphology and phase transition properties. J. Appl. Phys., 129, 185303(2021).
[39] K. Nishikawa, M. Yoshimura, Y. Watanabe. Phase transition behavior in nanostructured VO2 with M1, M2, and R phases observed via temperature-dependent XRD measurements. J. Vac. Sci. Technol. A, 40, 033401(2022).
[40] Q. Shi, W. Huang, T. Lu, Y. Zhang, F. Yue, S. Qiao, Y. Xiao. Nanostructured VO2 film with high transparency and enhanced switching ratio in THz range. Appl. Phys. Lett., 104, 071903(2014).
[41] H. Li, H. Djaoued, J. Robichaud, Y. Djaoued. A pleasant blue-green colored 2D vanadium dioxide inverse opal monolayer: large area fabrication and its thermochromic application. J. Mater. Chem. C, 8, 11572-11580(2020).
[42] W. Kou, W. Shi, Y. Zhang, Z. Yang, T. Chen, J. Gu, X. Zhang, Q. Shi, S. Liang, F. Lan, H. Zeng, Z. Yang. Terahertz switchable focusing planar lens with a nanoscale vanadium dioxide integrated metasurface. IEEE Trans. Terahertz Sci. Technol., 12, 13-22(2021).
[43] F. Ding, S. Zhong, S. I. Bozhevolnyi. Vanadium dioxide integrated metasurfaces with switchable functionalities at terahertz frequencies. Adv. Opt. Mater., 6, 1701204(2018).
[44] Y. Ren, B. Tang. Switchable multi-functional VO2-integrated metamaterial devices in the terahertz region. J. Lightwave Technol., 39, 5864-5868(2021).
[45] J. Huang, J. Li, Y. Yang, J. Li, J. Li, Y. Zhang, J. Yao. Active controllable dual broadband terahertz absorber based on hybrid metamaterials with vanadium dioxide. Opt. Express, 28, 7018-7027(2020).
[46] M. Mao, Y. Liang, R. Liang, L. Zhao, N. Xu, J. Guo, F. Wang, H. Meng, H. Liu, Z. Wei. Dynamically temperature-voltage controlled multifunctional device based on VO2 and graphene hybrid metamaterials: perfect absorber and highly efficient polarization converter. Nanomaterials, 9, 1101(2019).
[47] H. S. Choi, J. S. Ahn, J. H. Jung, T. W. Noh, D. H. Kim. Mid-infrared properties of a VO2 film near the metal-insulator transition. Phys. Rev. B, 54, 4621-4628(1996).
[48] C. Menzel, C. Helgert, C. Rockstuhl, E.-B. Kley, A. Tünnermann, T. Pertsch, F. Lederer. Asymmetric transmission of linearly polarized light at optical metamaterials. Phys. Rev. Lett., 104, 253902(2010).
[49] T. Wang, H. Zhang, Y. Zhang, Y. Zhang, M. Cao. Tunable bifunctional terahertz metamaterial device based on Dirac semimetals and vanadium dioxide. Opt. Express, 28, 17434-17448(2020).
[50] J. Zhao, J. Song, T. Xu, T. Yang, J. Zhou. Controllable linear asymmetric transmission and perfect polarization conversion in a terahertz hybrid metal-graphene metasurface. Opt. Express, 27, 9773-9781(2019).
[51] T. J. Cui, M. Q. Qi, X. Wan, J. Zhao, Q. Cheng. Coding metamaterials, digital metamaterials and programmable metamaterials. Light Sci. Appl., 3, e218(2014).
[52] F. Aieta, P. Genevet, M. A. Kats, N. Yu, R. Blanchard, Z. Gaburro, F. Capasso. Aberration-free ultrathin flat lenses and axicons at telecom wavelengths based on plasmonic metasurfaces. Nano Lett., 12, 4932-4936(2012).
[53] V. G. Golubev, V. Yu. Davydov, N. F. Kartenko, D. A. Kurdyukov, A. V. Medvedev, A. B. Pevtsov, A. V. Scherbakov, E. B. Shadrin. Phase transition-governed opal-VO2 photonic crystal. Appl. Phys. Lett., 79, 2127-2129(2001).
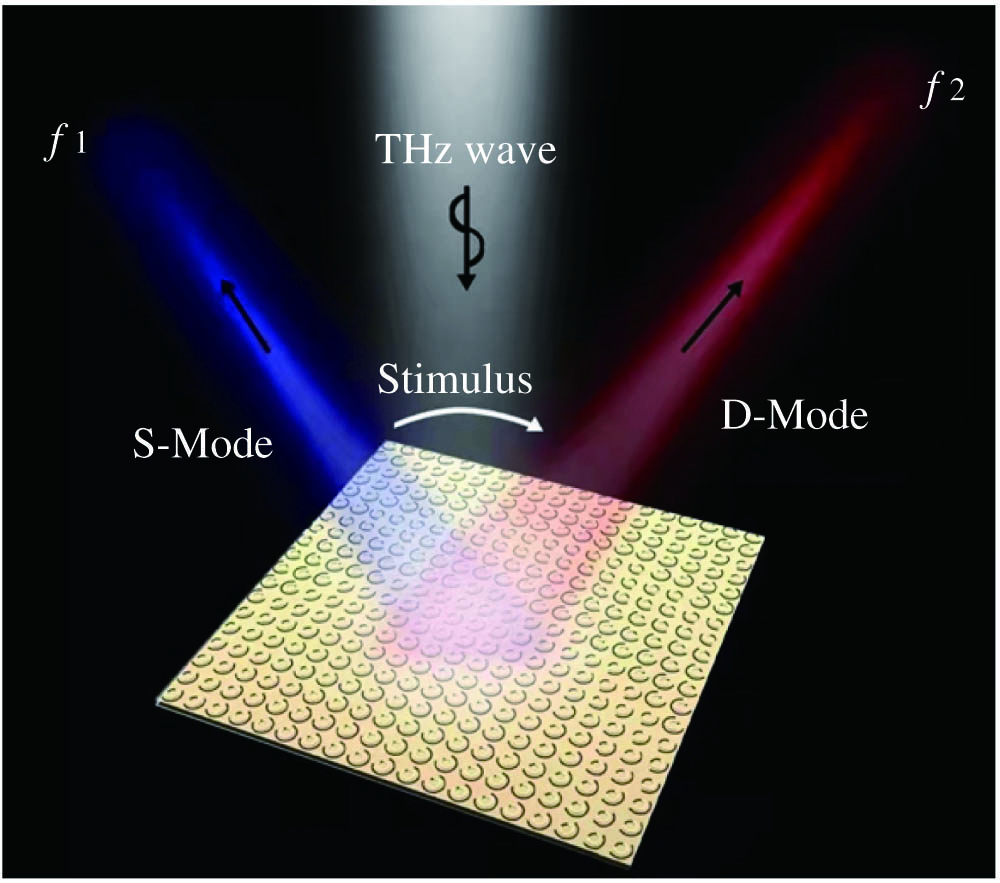
Set citation alerts for the article
Please enter your email address