
- Journal of Inorganic Materials
- Vol. 35, Issue 12, 1391 (2020)
Abstract
For decades, ultraviolet radiation receives rapidly growing attention due to its indispensable role in industrial production, security and anti-counterfeiting, health care, and other related fields[
Compared with traditional semiconductors, luminescent methods show clear advantages in high sensitivity, fast response rate, and low cost[
The 5f element uranium is an emerging element used as photosensitive and luminescent materials. The hexavalent uranium generally exists as the divalent linear dioxo unit of UO22+. The photoluminescence property of the uranyl unit originates from the HOMO-LUMO transition of hybridized molecular orbitals, which is not Laporte- forbidden, and hence the UV absorption efficiency and emission intensity of the uranyl often exceeds those of lanthanides[
Here, a bifunctional ligand containing both phosphinate and carboxylate moieties was utilized and a luminescent uranyl layered compound [(TEA)2(UO2)5(PhPC)6] (TEA = tetraethylammonium ion, PhPC = (2-carboxyethyl) phenylphosphinic acid, denoted as UPhPC-1) was synthesized via the hydrothermal method. The structure of UPhPC-1 was revealed by single crystal XRD technique, showing that both phosphinate and carboxylate groups are coordinated to the uranyl ions. The experimental results demonstrate that UPhPC-1 not only shows good thermal stability, hydrolytic stability, and radiation resistance, but also exhibits a fast response rate of 365 nm UV radiation with a low detection limit of 3.9 μJ. The relationship between the UV radiation dosage and the uranyl emission intensity was established and the fluorescence quenching mechanism is proposed to be a radical-induced quenching process. Further studies suggest that the radicals can be readily eliminated by heating, leading to the recovery of the photoluminescence intensity of UPhPC-1.
1 Experimental
1.1 Materials and synthesis
Materials (2-carboxyethyl)phenylphosphinic acid (98%, Energy Chemical) and tetraethylammonium hydroxide (25wt% solution in H2O, J&K Scientific) were used directly without further purification. While uranium compounds used in laboratories contain depleted uranium, standard procedures for handling radioactive materials should be followed.
Synthesis A mixture of UO2(NO3)2∙6H2O (0.05 g, 0.1 mmol), (2-carboxyethyl)phenylphosphinic acid (0.04 g, 0.2 mmol), tetraethylammonium hydroxide (0.2 mL), and H2O (2 mL) was added into a 20 mL Teflon-lined stainless steel autoclave. The autoclave was sealed and heated to 220 ℃ for 3 d, and then cooled to room temperature in 24 h. Brown yellow crystals were isolated as UPhPC-1.
1.2 Characterization
X-ray crystallography Single crystal X-ray diffraction data acquisition was carried out on a Bruker D8-Venture diffractometer with a Turbo X-ray Source (Mo-Kα radiation, λ=0.071073 nm) adopting the direct- drive rotating anode technique and a CMOS detector at 298 K. The data frames were collected using the program APEX3 and processed using SAINT routine in APEX3. The structure of UPhPC-1 was solved by direct methods and refined by the full-matrix least squares on F2 using the SHELXTL program.
Powder X-ray diffraction (PXRD) PXRD patterns were collected on a Bruker D8 Advance diffractometer at 40 kV and 40 mA with Cu Kα radiation (λ=0.154056 nm) and a Lynxeye one-dimensional detector from 5° to 50° with a step of 0.02° in 2θ.
Photoluminescence and UV-Vis absorption spectroscopy The crystals were placed on quartz slides and photoluminescence and UV-Vis absorption spectra were acquired after auto-set optimization by Craic Technologies microspectrophotometer. The photoluminescence spectra of the solid samples before and after X-ray irradiation were collected by Steady State & Transient State Fluorescence Spectrofluorometer.
Fourier transform infrared (FT-IR) spectroscopy The FT-IR spectra of the solid samples before and after irradiation were recorded in the range of 4000-400 cm-1 with the Thermo Nicolet iS 50 spectrometer.
Thermogravimetric analysis (TG) TG analysis was carried out using a NETZSCH STA449F3 instrument in the temperature range from 30-900 ℃ under a nitrogen flow at a heating rate of 10 K/min for the dried sample.
Elemental analysis Elemental analysis (C, H, and N) was performed with a Vario EL CHNOS elemental analyzer.
Electron paramagnetic resonance (EPR) spectroscopy The EPR data of the pristine sample and samples irradiated for 90 min by UV light and 226 s by X-ray were recorded on a Bruker EMXplus 10/12 EPR spectrometer equipped with an Oxford Instruments EPR901 liquid helium continuous-flow cryostat fitted with a super- high-Q cavity, respectively.
UV detection experiments UV detection studies were carried out and luminescence spectra were recorded at various exposure time under 365 nm light.
X-ray irradiation experiments The experiments were carried out using a RS-2000 Pro Biological Irradiator equipped with Cu Kα radiation at a dose rate of 26.5 Gy/min and the samples were irradiated for 226 s.
Hydrolytic stability measurements The hydrolytic stability evaluation of UPhPC-1 was performed by soaking the samples in HNO3/NaOH aqueous solutions in the pH range from 5 to 12 and shaking for 24 h. The soaked samples were isolated and dried for PXRD pattern analysis.
2 Results and discussion
2.1 Structural elucidation
Single crystal X-ray diffraction analysis reveals that UPhPC-1 crystallizes in the centrosymmetric space group, P$\overline 1$. As shown in Fig. 1 and Table S1, the asymmetric unit consists of two and a half unique uranyl ions, three PhPC ligands, and one distinctive tetraethylammonium ion.
Figure 1.Structural depiction of UPhPC-1(a) View of the layer topology in the [
Formula | [N(C2H5)4]2(UO2)5[C6H5PO2C2COO]6 |
---|---|
Mr/(g•mol-1) | 2883.45 |
Color and habit | Yellow, block |
Crystal system | Triclinic |
Space group | $P \overline 1$ |
1.0802(4) | |
1.2288(5) | |
1.7544(5) | |
86.856(13) | |
78.009(10) | |
86.530(18) | |
2.2714(15) | |
1 | |
2.108 | |
298 | |
0.0322 | |
0.0816 | |
a | b |
Table 1.
Crystallographic data of UPhPC-1 (CCDC No. 1978001)
The U1 and U3 units are in the pentagonal bipyramidal geometry with axial U=O bond distances ranging from 0.1781(5) to 0.1789(4) nm. The U2 unit sits at the inversion center and is in tetragonal bipyramidal geometry with the typical uranyl bond distance of 0.1777(4) nm. All equatorial-bonding oxygen atoms of three uranyl units are provided from the PhPC ligand with bond distances ranging from 0.2309(5) to 0.2491(4) nm. The bond valence sum values of the U1, U2, and U3 are calculated to be 6.019, 5.739, and 6.094, respectively. As shown in Fig. 1, all uranyl units are connected by the PhPC ligands to form the uranyl layers, which are packed via hydrogen bonding networks and π-π interactions to yield the overall layered structure. In the free space, there are tetraethylammonium cations to compensate the negative charge of the uranyl layers. The formula of UPhPC-1 is determined to be (TEA)2(UO2)5(PhPC)6 taken the results of single crystal structural analysis and elemental analysis (Table S2) into consideration. The FT-IR spectrum of UPhPC-1 was collected without KBr at room temperature. As shown in Fig. S1, the characteristic vibration peaks of the phenyl rings and the carboxylate units are identified between 1720 and 1250 cm-1[
Content/% | Peak area | Daily factor | |
---|---|---|---|
N | 0.0832 | 929 | 0.9751 |
C | 28.68 | 20169 | 0.9846 |
H | 3.409 | 6670 | 0.9800 |
Table 2.
The elemental analyses of UPhPC-1
Figure S1.FT-IR spectra of the pristine UPhPC-1 (a), and UPhPC-1 after UV irradiation and 100 Gy X-ray irradiation (b)
2.2 Irradiation dosage dependent luminescence spectra
The photoluminescence spectrum of UPhPC-1 was collected under 365 nm excitation light at room temperature. As shown in Fig. S2, the spectrum of UPhPC-1 exhibits the characteristic emission peaks at 495, 516, 539, 565, and 592 nm, corresponding to the S10-S0n (n = 0-4) transitions of the uranyl unit. Compared with the emissive spectrum of UO2(NO3)2·6H2O, the peak position of UPhPC-1 was red-shifted by ca. 7 nm, probably caused by the coordination of the uranyl unit and the PhPC ligand, which is generally observed in uranyl coordination polymers[
Figure S2.Photoluminescent spectra of UPhPC-1 (
Figure S3.UV-Vis absorption spectrum of UPhPC-1 at 298 K
To our surprise, the luminescent emission of UPhPC-1 was quenched rapidly after UV light irradiation, which could be observed by naked eyes. This phenomenon strongly suggests the potential of this material being used as a dosimeter for ultraviolet radiation. As shown in Fig. 2, the emission intensity of UPhPC-1 decreased significantly with the accumulative increase of the UV radiation dosage, indicating that the luminescence intensity of UPhPC-1 is responsive to the dosage of UV light not only instantly but also accumulatively. The emission peak intensity of UPhPC-1 was decreased by 79% after being exposed to 0.84 mJ 365 nm radiation and was almost completely quenched after 1.5 h exposure (Fig. 2(a)). To quantitively describe the negative correlation between luminescence intensity and UV radiation dosage, the quenching ratio of UPhPC-1 was calculated and expressed as (I0-I)/I0, where I0 is the initial luminescence intensity and I is the luminescence intensity after irradiation by ultraviolet light. The detection limit of UPhPC-1 can be determined by the following equations:
Figure 2.Luminescence spectra (a) of UPhPC-1 with increasing doses of UV radiation, correlation between the quenching ratio and the UV radiation dose (b) (measured at 516 nm), and corresponding photographs (c) of the single crystal under continuous UV irradiationThe inset is the linear fitting of the point data in the low dose range (0-0.028 mJ)
Here DT is the detection limit; ISE is the standard error of the luminescence intensity; I0 is the measured initial luminescence intensity of UPhPC-1; k is the slope obtained from the linear fit in the low dosage range of the dosage-dependent luminescence intensity calibration curve.
The linear fitting can be achieved for the curve of the quenching ratio versus the dosage of UV irradiation in the low dose range (Fig. 2(b)). The detection limit of UPhPC-1 was determined to be 3.9 μJ. Besides, the response rate of UPhPC-1 is faster than the uranyl-oxalate based UV detection material, demonstrating the high sensitivity of UPhPC-1 for the low dosage ultraviolet radiation. When exposed with 100 Gy X-ray, the luminescence intensity of UPhPC-1 was quenched by 66% (Fig. 3(a)).
Figure 3.Photoluminescence spectra (a) of UPhPC-1 before and after X-ray irradiation, EPR spectra (b) of UPhPC-1 before and after UV and X-ray irradiation, luminescence intensities (c) of UPhPC-1 before and after UV irradiation, and after the heating recovery process
2.3 Stability evaluation
The stability of UPhPC-1 was investigated in this work. The PXRD and FT-IR data show that the structural integrity of UPhPC-1 was maintained after UV light and X-ray irradiation without any light-induced crystal degradation (Fig. S1 and Fig. S4). Those results demonstrate the high radiation resistance of UPhPC-1, which is comparable to other uranyl-based detection materials. The hydrolytic stability of UPhPC-1 was evaluated by soaking the crystalline samples in HNO3/NaOH aqueous solutions in the pH range from 5 to 12 and shaking for 24 h. The experimental PXRD patterns of the soaked samples match well with the pattern of the pristine UPhPC-1 sample, indicating the excellent hydrolytic stability of UPhPC-1 (Fig. S4). Thermogravimetric analysis reveals that UPhPC-1 experienced several stages of weight loss in the temperature range from 30 to 900 ℃. As shown in Fig. S5, the first weight-loss of 1.72% before 380 ℃ is assigned to the loss of surface water molecules during the heating process. The loss of tetraethylammonium ion occurred at temperature above 400 ℃, resulting in the 10.7% loss in weight and the disruption of the overall structure. This decomposition temperature is higher than that of a previous reported two-dimensional uranyl-oxalate compound of 306 ℃[
Figure S4.PXRD patterns of samples irradiated by UV and X-ray radiation and soaking in HNO3/NaOH aqueous solutions at pH range from 5 to 12 for 24 h
Figure S5.Thermogravimetric analysis curve of UPhPC-1
2.4 Quenching mechanism and reusability investigation
The EPR analyses were performed on the irradiated and pristine crystalline samples to further investigate the fluorescence quenching mechanism (Fig. 3(b)). It was observed that the peak intensity of the irradiated samples increased significantly compared to the original sample, implying the generation of radicals, yet further theoretical calculation is necessary to identify those radicals. On the basis of the obtained data, we speculate that the most plausible quenching mechanism is the production of radicals possibly from the partial bond break of the ligand in the irradiated crystal, resulting in the energy transfer from the uranyl center to the nearby generated radicals, which leads to the quenching of the luminescence of UPhPC-1[
The reusability of UV detection materials is also critical for their industrial development. Generally, it is not easy to obtain a reusable uranyl-based UV detection material since the recovery of the material requires not only the easy elimination of irradiation induced radicals, but also the ability to maintain the structural integrity during the irradiation and the recovery processes to restore its original photophysical properties. Generally, free radicals will not be able to stable for a long time, and heating accelerates the quenching process. The thermal elimination of radicals has been widely studied ever since 1970’s and has already been applied in the industrial production[
3 Conclusion
In conclusion, we herein report a novel uranyl layered compound UPhPC-1 with high thermal and hydrolytic stability and radiation resistance. The intrinsic luminescence of UPhPC-1 was found to be highly sensitive to UV and 100 Gy X-ray irradiation, and a negative correlation between the emission intensity and the irradiation dosage was established with a low detection limit. The EPR data indicate that the production of free radicals after irradiation is responsible for the quenching of the uranyl emission. Therefore, we can reasonably conclude that UPhPC-1 exhibits suitable application potential in the field of quantitative UV radiation detection and provides a new application platform for a large inventory of depleted uranium.
Supporting Materials
Supporting materials related to this article can be found at https://doi.org/10.15541/jim20200139.
References
[1] TORRES S GINER, N MONTANES, O FENOLLAR et al. Development and optimization of renewable vinyl plastisol/wood flour composites exposed to ultraviolet radiation. Mater. Design, 108, 648-658(2016).
[8] G GILBERT FRITZ, S KENT WOOD, VAN DEBORAH VECHTEN et al. Thermoelectric single-photon detectors for X-ray/UV radiation. X-Ray and Gamma-Ray Instrumentation for Astronomy XI. International Society for Optics and Photonics, 4140, 459-469(2000).
[35] TAO ZHENG, ZAI-XING YANG, DA-XIANG GUI et al. Overcoming the crystallization and designability issues in the ultrastable zirconium phosphonate framework system. Nat. Commun, 8, 15369(2017).
[37] TAO TIAN, WEI-TING YANG, ZHONG-MING SUN et al. Syntheses and structures of uranyl ethylenediphosphonates: from layers to elliptical nanochannels. Inorg. Chem, 52, 7100-7106(2013).
[38] WEI-TING YANG, WAN-GUO TIAN, ZHONG-MING SUN et al. Syntheses, structures, luminescence, and photocatalytic properties of a series of uranyl coordination polymers. Cryst. Growth Des, 14, 5904-5911(2014).
[39] WEI-TING YANG, GANNON T PARKER, ZHONG-MING SUN. Structural chemistry of uranium phosphonates. Coord. Chem. Rev, 303, 86-109(2015).
[40] WEN-TING LAN, LI HE, YAO-WEN LIU. Preparation and properties of sodium carboxymethyl cellulose/sodium alginate/chitosan composite film. Coatings, 8, 291(2018).
[42] IDA POLJANŠEK, MATJAŽ KRAJNC. Characterization of phenol- formaldehyde prepolymer resins by in line FT-IR spectroscopy. Acta. Chim. Slov, 52, 238-244(2005).
[44] ANDREEA IRINA COZACIUC, RODICA POSTOLACHI, ROBERT GRADINARU et al. Synthesis and characterization of uranyl (VI) chiral Schiff-base complexes derived from salicylaldehyde and L-aminoacids. J. Coord. Chem, 65, 2170-2181(2012).
[45] WEI-TING YANG, HAO WANG, ZHONG-MING SUN et al. The first family of actinide carboxyphosphinates: two and three- dimensional uranyl coordination polymers. Eur. J. Inorg. Chem, 5378-5384(2014).
[47] WEI-TING YANG, HAO WANG, ZHONG-MING SUN et al. Isolation of a series of uranium organophosphinates. Cryst. Eng. Comm, 16, 8073-8080(2014).
[49] PETERCA RSTENSEN. Free radicals in diene polymers induced by ultraviolet irradiation. II. an ESR study of cis-1.4-poly(butadiene). Die Makromolekulare Chemie: Macromol. Chem. and Phys, 142, 131-144(1971).
[50] XIAN ZHANG, SHENG-MING WANG, CAI SUN et al. Stabilizing and color tuning pyrazine radicals by coordination for photochromism. Chem. Commun, 52, 7947-7949(2016).
[51] SHENG-MING WANG, GANG XU, ZHANGJ-ING ZHANG et al. Inorganic-organic hybrid photochromic materials. Chem. Commun, 46, 361-376(2010).
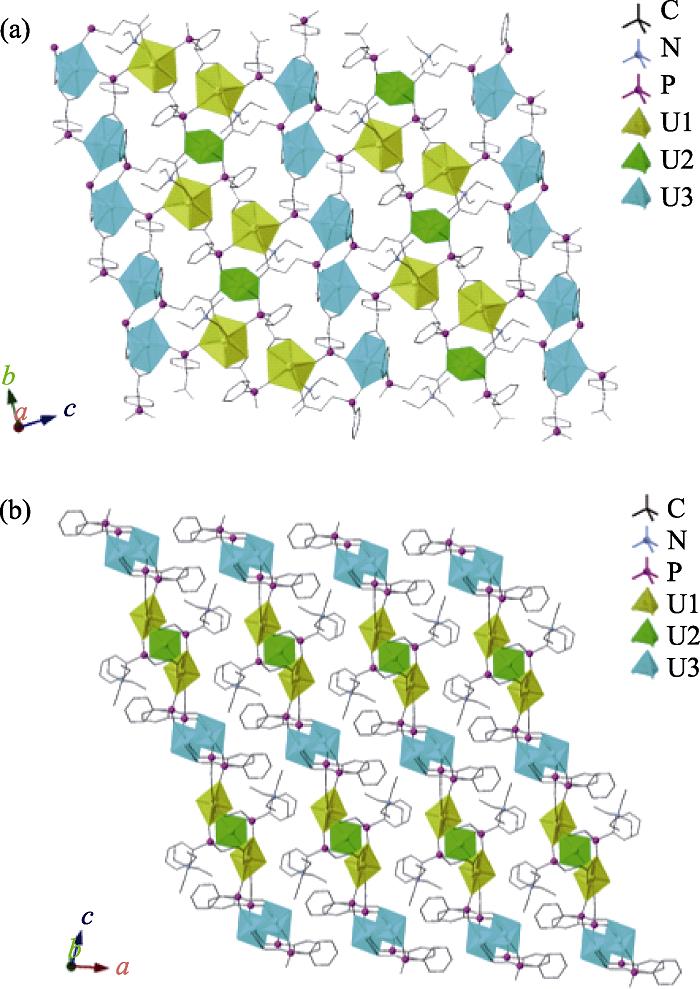
Set citation alerts for the article
Please enter your email address