
- Chinese Optics Letters
- Vol. 19, Issue 12, 123603 (2021)
Abstract
1. Introduction
Now, the human life has a big challenge to fight against the pandemic provoked by a coronavirus (COVID-19); for this reason, research works in the biosensing field are very developed now. Starting by the definition of a biosensor, which is proposed by the International Union of Pure and Applied Chemistry (IUPAC) as “an electrochemical biosensor is a self-contained integrated device, which is capable of providing specific quantitative or semi-quantitative analytical information using a biological recognition element (biochemical receptor) which is retained in direct spatial contact with an electrochemical transduction element”[
2. Design of the Proposed Biosensor
The demand for realization of an optical biosensor for molecule detection is becoming an urgent need to be satisfied to meet the direct real time and free detection of many viruses and biological and chemical substances. It is worth noting that a biosensor in the nm scale based on graphene/MNPs material is a device incorporating a biological sensing element used for the production of the concentration/nature of molecules proportional to measured signals[
Figure 1.Schematic of the studied optical device based on the Ag MNPs chain-graphene-Au MNPs chain, where vibrational molecules are placed on the surface of graphene lattice. The nanoelectrodes of our device formed by two parallel linear chains of (A) Ag MNPs and (B) Au MNPs are separated by a distance (h) and incorporated in a suspended graphene single layer. We shine only the Ag chain (A) by an external light beam, and the output detector is placed at the end of the Au chain (B).
In practice, the optical response of the Ag/Au-MNPs-loaded graphene sheet was measured by injecting a polarized laser light at the Ag MNPs entrance chain (A). In order to control the incident beam to hit only the Ag chain, a spot size converter device (SSC) with an output signal around 80 nm can be used to couple the laser polarized light to the entrance facet of Ag MNPs. The light at the end of the Au MNPs chain was collected by an objective and focused onto a power meter. Ag MNPs have great potential in a broad range of applications, such as in biomedical device coatings. It is expected that Ag nanoparticles can be easily prevented from oxidation by providing suitable surfactant as a protection barrier[
Sign up for Chinese Optics Letters TOC. Get the latest issue of Chinese Optics Letters delivered right to you!Sign up now
As mentioned above, precise control over the geometry of these nanoparticles is nontrivial, and ion beam shaping is a new method used to engineer nanocomposites with effective three-dimensional (3D) architectures. In particular, this technique offers the possibility to precisely control the size, shape, and 3D orientation of MNPs at the nm scale placed at the vicinity of a graphene sheet[
Ag | Au | |
---|---|---|
ωp (Hz) | ||
Table 1. Plasma Frequency
3. Results and Discussion
3.1. Electrical and optical properties of graphene-molecules hybrid system
We propose to take advantage of the electrical properties of graphene, so the proposed nanospheres are incorporated in a graphene lattice, which is considered a receptor of our biosensor, where the vibrational molecules to be detected are placed in its vicinity. Graphene is a 2D sheet of carbons with an atomic thickness that exhibits very important electrical, optical, and thermal properties. It contains free carriers (electrons) with high mobility. Despite being only one atom thick, graphene absorbs a significant fraction of light, so there is very important coupling with detected molecules. The dispersion relation of graphene shows that graphene can be considered as a semi-conductor with zero gap, which is because graphene is able to generate SP modes like metals propagating with high speed. Here, all electrical properties of graphene are summarized in its conductivity
Figure 2.Variation of the real part of the permittivity of the graphene-molecules hybrid system for molecule concentrations S = 0 nm−3, S = 0.01 nm−3, and S = 0.014 nm−3, where the chemical potential of graphene is μ = 0.21 eV. (a) ℏωm = 280 meV and (b) ℏωm = 284 meV.
For the two selected molecules that are characterized by
3.2. Performance of the LSP/SP/LSP biosensor
Let us investigate firstly where only the Ag chain (A) is incorporated in graphene. Using the point dipole approximation, the generation of the LSPs in the Ag chain can be modeled via the dipole-dipole interaction (DDI). Here, the involved induced dipole is calculated using the iterative equation:
Here,
Combining Eqs. (6), (8) and (9), the polarization in
Now, in order to discuss the effect of the chemical potential
Figure 3.Transmission coefficient (T) as a function of frequency for the three selected molecules that are characterized by ℏωm = 280 meV, ℏωm = 284 meV, and ℏωm = 288 meV, respectively. The chemical potential of the graphene layer is equal to 0.21 eV at room temperature.
Figure 4.Transmission coefficient (T) as a function of frequency for the three selected molecules characterized by ℏωm = 280 meV, ℏωm = 284 meV, and ℏωm = 288 meV, respectively. The chemical potential of the graphene layer is equal to 1.21 eV at room temperature.
As mentioned above, we found that the origin of the variation in the transmission spectra is mainly coming from the presence of the molecules that affect the density of defect in the graphene superlattice. Generally, the presence of molecules changes the figures of merit (FOMs) of devices, such as carrier mobility and conductivity[
For graphene at room temperature, it is clearly noticeable from Fig. 5 that the presence of the molecules near the graphene surface strongly affects the transmission in the Au MNPs chain. As shown in Fig. 5 with
Figure 5.Transmission spectra calculated at the end of chain (B) with and without molecules, and the chemical potential of graphene is μ = 1.21 eV.
Figure 6.Transmission spectra for different value of h when ωm = 280 meV and μ = 1.21 eV.
It is worth noting that molecule detection is controlled by the nonlinear conductivity of graphene, so, by activating the graphene monolayer (i.e., varying chemical potential
Figure 7.Transmission spectrum of light passing through the second chain (B) for the two values of the chemical potential μ = 0.21 eV and μ = 1.21 eV.
In Figs. 8(a) and 8(b), we plot the absorption spectra of the graphene-molecules system, which is calculated via the equation
Figure 8.Variation of the absorption in graphene (red line), transmission of light passing through Ag chain (red dashed line), and transmission at the end of the Au chain (black line) for (a) ℏωm = 280 meV and (b) ℏωm = 284 meV when the chemical potential μ = 0.21 eV.
4. Conclusion
The coupling LSP-SPP-LSP modes at the nanoscales are achieved in order to realize a nanobiosensor device based on Ag MNPs-graphene-Au MNPs. In this work, we demonstrate that the nonlinear properties of the graphene sheet could play a major role in realizing a biosensor for molecule detection. It is established that the chemical potential
References
[1] D. R. Thévenot, K. Toth, R. A. Durst, G. S. Wilson. Electrochemical biosensors: recommended definitions and classification. Biosens. Bioelectron., 16, 121(2001).
[2] P. Damborský, J. Švitel, J. Katrlík. Optical biosensors. Essays Biochem., 60, 91(2016).
[3] S. M. Yoo, S. Y. Lee. Optical biosensors for the detection of pathogenic microorganisms. Trends Biotechnol., 34, 7(2016).
[4] C. Chen, J. Wang. Optical biosensors: an exhaustive and comprehensive review. Analyst, 145, 1605(2020).
[5] K. Xu, Y. Chen, T. A. Okhai, L. W. Snyman. Micro optical sensors based on avalanching silicon light-emitting devices monolithically integrated on chips. Opt. Mater. Express, 9, 3985(2019).
[6] K. Xu. Silicon electro-optic micro-modulator fabricated in standard CMOS technology as components for all silicon monolithic integrated optoelectronic systems. J. Micromech. Microeng., 31, 054001(2021).
[7] M. Soler, O. Calvo-Lozano, M.-C. Estevez, L. M. Lechuga. Nanophotonic biosensors: driving personalized medicine. Opt. Photon. News, 31, 4(2020).
[8] M. Mesch, B. Metzger, M. Hentschel, H. Giessen. Nonlinear plasmonic sensing. Nano Lett., 16, 3155(2016).
[9] G. Emir, Y. Dilgin, A. Ramanaviciene, A. Ramanavicius. Amperometric nonenzymatic glucose biosensor based on graphite rod electrode modified by Ni-nanoparticle/polypyrrole composite. Microchem. J., 161, 105751(2021).
[10] Y. Zhou, A. Marar, P. Kner, R. P. Ramasamy. Charge-directed immobilization of bacteriophage on nanostructured electrode for whole-cell electrochemical biosensors. Anal. Chem., 89, 5734(2017).
[11] A. B. Socorro-Leránoz, D. Santano, I. Del Villar, I. R. Matias. Trends in the design of wavelength-based optical fibre biosensors (2008–2018). Biosens. Bioelectron. X, 1, 100015(2019).
[12] D. T. Hoa, T. N. Suresh Kumar, N. S. Punekar, R. S. Srinivasa, R. Lal, A. Q. Contractor. Biosensor based on conducting polymers. Anal. Chem., 64, 2645(1992).
[13] E. A. Chiticaru, L. Pilan, C. M. Damian, E. Vasile, J. S. Burns, M. Ioniţă. Influence of graphene oxide concentration when fabricating an electrochemical biosensor for DNA detection. Biosensors, 9, 113(2019).
[14] J. Peña-Bahamonde, H. N. Nguyen, S. K. Fanourakis. Recent advances in graphene-based biosensor technology with applications in life sciences. J. Nanobiotechnol., 16, 75(2018).
[15] S. Liu, Z. Yang, Y. Chang, Y. Chai, R. Yuan. An enzyme-free electrochemical biosensor combining target recycling with Fe3O4/CeO2 Au nanocatalysts for microRNA-21 detection. Biosens. Bioelectron., 119, 170(2018).
[16] C. Liu, X. Zeng, Z. An, Y. Yang, Y. Yang, M. Eisenbaum, X. Gu, J. M. Jornet, G. K. Dy, M. E. Reid, Q. Gan, Y. Wu. Sensitive detection of exosomal proteins via a compact surface plasmon resonance biosensor for cancer diagnosis. ACS Sens., 3, 1471(2018).
[17] Y. Yi, B. Xie, T. Zhao, Z. Li, D. Stom, H. Liu. Effect of external resistance on the sensitivity of microbial fuel cell biosensor for detection of different types of pollutants. Bioelectrochemistry, 125, 71(2019).
[18] S. Campuzano, P. Yáñez-Sedeño, J. M. Pingarrón. Molecular biosensors for electrochemical detection of infectious pathogens in liquid biopsies: current trends and challenges. Sensors, 17, 2533(2017).
[19] M. Manzano, S. Viezzi, S. Mazerat, R. S. Marks, J. Vidic. Rapid and label-free electrochemical DNA biosensor for detecting hepatitis A virus. Biosens. Bioelectron., 100, 89(2018).
[20] M. Manzano, S. Viezzi, S. Mazerat, R. S. Marks, J. Vidic. Rapid and label-free electrochemical DNA biosensor for detecting hepatitis A virus. Biosens. Bioelectron., 100, 89(2018).
[21] N. Dasgupta, H. V. Raghu, T. Parkunan, S. Ranjan, N. Kumar, E. Lichtfouse. Application of nanobiosensors for food safety monitoring. Environmental Nanotechnology(2020).
[22] L. Wu, H. S. Chu, W. S. Koh, E. P. Li. Highly sensitive graphene biosensors based on surface plasmon resonance. Opt. Express, 18, 14395(2010).
[23] K. Saha, S. S. Agasti, C. Kim, X. Li, V. M. Rotello. Gold nanoparticles in chemical and biological sensing. Chem. Rev., 112, 2739(2012).
[24] J. Meyer, A. Geim, M. Katsnelson. The structure of suspended graphene sheets. Nature, 446, 60(2007).
[25] L. Pu, M. Baig, V. Maheshwari. Nanoparticle chains as electrochemical sensors and electrodes. Anal. Bioanal. Chem., 408, 2697(2016).
[26] X. Zhao, L. Yang, J. Guo, T. Xiao, Y. Zhou, Y. Zhang, B. Tu, T. Li, B. A. Grzybowski, Y. Yan. Transistor and logic circuit based on metal nanoparticles and ionic gradients. Nat. Electron., 4, 109(2021).
[27] S. H. Lee, B.-H. Jun. Silver nanoparticles: synthesis and application for nanomedicine. Int. J. Mol. Sci., 20, 865(2019).
[28] L. J. Mendoza Herrera, D. M. Arboleda, D. C. Schinca, L. B. Scaffardi. Determination of plasma frequency, damping constant, and size distribution from the complex dielectric function of noble metal nanoparticles. J. Appl. Phys., 116, 233105(2014).
[29] P. E. Coulon, J. Amici, M. C. Clochard. Ion-shaping of embedded gold hollow nanoshells into vertically aligned prolate morphologies. Sci. Rep., 6, 21116(2016).
[30] Y. Gao, I. Shadrivov. Nonlinear coupling in graphene-coated nanowires. Sci. Rep., 6, 38924(2016).
[31] L. Zundel, A. Manjavacas. Spatially resolved optical sensing using graphene nanodisk arrays. ACS Photon., 4, 1831(2017).
[32] N. A. Savostianova, S. A. Mikhailov. Optical Kerr effect in graphene: theoretical analysis of the optical heterodyne detection technique. Phys. Rev. B, 97, 165424(2018).
[33] S. Sumi, H. Awano, M. Hayashi. Interference induced enhancement of magneto-optical Kerr effect in ultrathin magnetic films. Sci. Rep., 8, 776(2018).
[34] H. Nadjari, Z. Abasi Azad. Determining the nonlinear coefficient of gold and silver nano-colloids using SPM and CW Z-scan. Opt. Laser Technol., 44, 1629(2012).
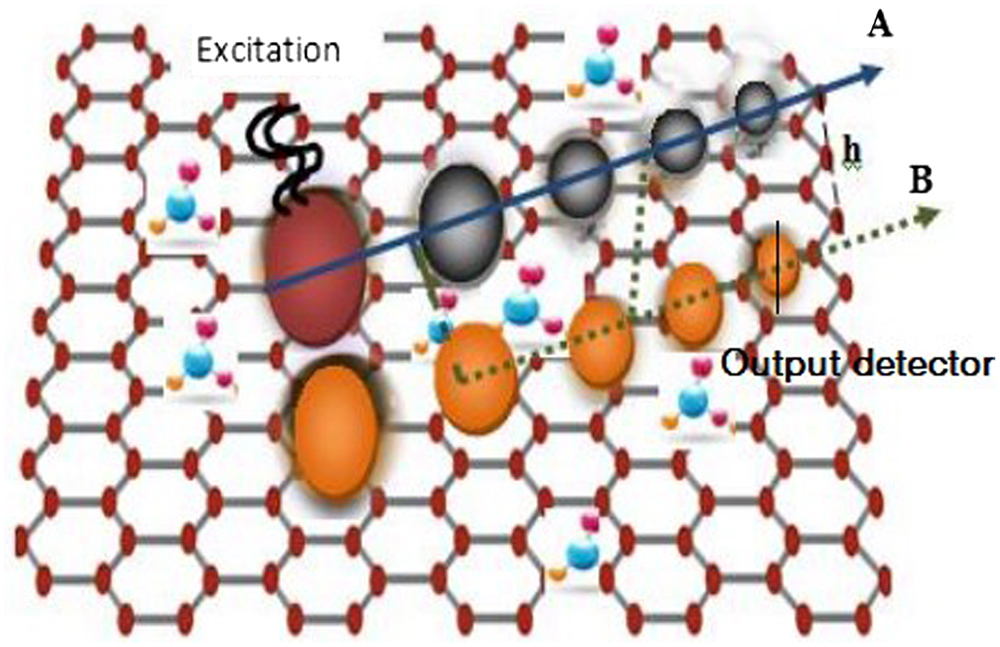
Set citation alerts for the article
Please enter your email address