
- Journal of Inorganic Materials
- Vol. 36, Issue 3, 325 (2021)
Abstract
The emergence of semiconductor-based photocatalysis has drawn increasing interest because of its universal applications in environmental remediation and solar energy utilization[
To solve the problem mentioned above, noble metal loading is one of commonly used approaches to achieve better charge separation for efficient photocatalysis[
Recently, much attention has been paid to promoting the photocatalytic activity of cube-like WO3 nanostructure by coupling with noble metal or other semiconductors[
1 Experimental section
1.1 Materials synthesis
In synthesis of WO3 nanocube, 3.0 g Na2WO4·2H2O was firstly dissolved in 65 mL H2O. Then, 15 mL HCl (37%) was added into the solution gradually under vigorous magnetic stirring for 30 min. The resulting suspension was transferred to a 100 mL Teflon-lined autoclave and maintained at 180 ℃ for 10 h. After cooling down to room temperature, the precipitant was centrifuged and washed thoroughly with deionized water and ethanol for several times, and finally was dried at 60 ℃ overnight[
In fabrication of WO3-Cu hybrid, 0.2 g WO3 was firstly put into a round bottle containing 10 mL deionized water and 10 mL ethylene glycol. After sonication, 0.03 mmol CuSO4·5H2O was dissolved into the solution under sonication. Then, 10 mL 0.5 mol/L ascorbic acid aqueous solution was gradually dropped into the above solution and further stirred for 2 h. Finally, the solid product was centrifuged and washed thoroughly with deionized water and ethanol for several times, and was dried at 60 ℃ overnight. This sample was denoted as WO3-Cu-0.03. When 0.08, 0.3, 1.0, 3.0 mmol CuSO4·5H2O was involved into the reaction system, the sample was denoted as WO3-Cu-0.08, WO3-Cu-0.3, WO3-Cu-1.0, WO3-Cu-3.0, respectively.
1.2 Characterizations
X-ray powder diffraction (XRD) was carried out on a Bruker D8 Advance diffractometer by using Cu Kα radiation at a scan rate of 10 (°)/min in the 2θ range from 10° to 80°. Scanning electron microscopy (SEM) image was conducted on a JSM 5510LV at the operating voltage of 5 kV. The energy-dispersive X-ray spectrum (EDX) analysis was carried out on an Oxford Instrument INCA with a scanning range from 0 to 20 kV. Transmission electron microscopy (TEM) images were visualized on a JEM-2000, using an accelerating voltage of 200 kV. The Electron Spin Resonance (ESR) was performed on a Hitachi ESR spectrometer (JES, FA200). The transient photocurrent density and electrochemical impedance spectra (EIS) were analyzed on a CHI660E electrochemical workstation with a standard three-electrode system according to previous study[
1.3 Photocatalysis test
In a typical photocatalysis test, 0.02 g catalyst was added into a reactor containing CR solution (40 mL, 20 mg/L) and sonicated to get homogeneous solution. Then, it was stirred for 1 h in the dark to reach adsorption-desorption equilibrium. Subsequently, the reactor with WO3-Cu catalyst and CR solution was exposed to simulated sun light irradiation by a 500 W Xe lamp with magnetically stirring. 3 mL of the suspensions at each irradiation time interval was collected and then centrifuged. The collected upper solution was analyzed by a Shimadzu UV2800 spectrophotometer.
2 Results and discussion
Fig. 1(a) shows the photographs of the as-synthesized WO3-Cu samples. It can be observed that the color gradually deepens with the increase of Cu content. As shown in Fig. 1(b), all diffraction peaks of the samples can be assigned with the standard pattern of WO3 (JCPDS 72-677), indicating that the hybrid contained the main phase of WO3. The symbol of ★ corresponds to the Cu species in the XRD patterns. With Cu amounts increasing, the diffraction peak of Cu occurred and the intensity became stronger. This result suggested that WO3-Cu hybrid be successfully prepared through the ascorbic acid reduction method at room temperature.
Figure 1.(a) Photographs and (b) XRD-patterns of WO3-Cu samples
As shown in Fig. 2(a), the UV-Vis diffuse reflectance spectra demonstrate that the WO3 nanocube have absorption edge in the visible light region. Compared to pure WO3 product, a red shift to higher wavelength in the absorption edge of WO3-Cu hybrids was observed. Fig. 2(b) reveals the band gap of the pure WO3 is about 2.49 eV. With the content of Cu increasing, the obtained WO3-Cu sample showed a narrow band gap. This result further confirms that the WO3-Cu hybrids are successfully synthesized. The valence band (EVB) and conduction band (ECB) edge of WO3 sample at the point of zero charge can be calculated by the empirical equation EVB= X-Ee+0.5Eg, where X is the electronegativity of the semiconductor, Ee is the energy of free electrons on the hydrogen scale (about 4.5 eV), Eg is the band gap energy of the semiconductor, and ECB can be determined by ECB=EVB-Eg[
Figure 2.(a) UV-Vis diffuse reflectance spectra (DRS) and (b) plots of (
As depicted in Fig. 3(a, b), the WO3-Cu-1.0 hybrid exhibited the same morphology with pure WO3. The EDX-spectrum (Fig. 3(c)) was also performed to analyze the compositions of the WO3-Cu-1.0 hybrid. It illustrates that the sample is composed of W, O and Cu, which is in good agreement with the XRD result. The structure of the WO3-Cu-1.0 hybrid was further confirmed by TEM images. As revealed in Fig. 3(d, e), the Cu particle was deposited on the surface of WO3 nanocube in the hybrid. Fig. 3(e) displays the HRTEM image of an individual WO3 nanocube decorated with Cu particle. The clear lattice fringes of 0.37 and 0.38 nm, which correspond to the (020) and (002) crystal planes of WO3, respectively. On the surface of WO3 nanocube, the lattice d-spacing of 0.21 nm assigned well with the (111) crystal plane of Cu. In addition, as shown in Fig. 3(g, i), Cu, O, W elements were found in terms of the selected area. This result also indicated that the hybrids of Cu particles deposited on WO3 nanocubes were successfully obtained.
Figure 3.SEM images of (a) WO3 and (b) WO3-Cu-1.0 sample, (c) EDX spectrum of WO3-Cu-1.0 sample, (d) TEM and (e) HRTEM images of WO3-Cu-1.0 sample, and (f-i) EDX mappings of WO3-Cu-1.0 sample
Fig. 4(a) shows the variation in Congo Red (CR) concentration (C/C0) versus irradiation time upon different samples under simulated sun light irradiation. It was found that the WO3-Cu hybrid displayed superior photocatalytic performance than pure WO3, while the Congo Red cannot be removed by direct photolysis process. Furthermore, the WO3-Cu-1.0 exhibited the best performance among the hybrids, and it could remove 85% Congo Red from the solution. Fig. 4(b) shows a pseudo- first-order model, ln(C0/Ct)=kt (where k is the pseudo- first-order constant, t is the reaction time). To understand the reaction kinetics of the CR photodegradation, the k value was listed in Fig. 4(c). It was observed that the WO3-Cu-1.0 had the highest k, indicating its good photocatalytic activity. In addition, Fig. 4(d) indicates that the WO3-Cu-1.0 also owns good cycling photodegradation performance.
Figure 4.(a) Photocatalytic performance and (b) CR degradation reaction dynamics of different samples, (c) pseudo-first-order constant
Fig. 5(a) shows the influence of the addition of the scavengers, including methanol, isopropanol, CCl4, and N2, for h+, •OH, electron, and •O2- removal, respectively, on the related photocatalytic property. It was found that the CR photodegradation decreased with involving of methanol, isopropanol, or N2, while the introduction of CCl4 had no impact on the photocatalysis. It was proposed that the generated h+, •OH and •O2- were the predominant active species during the photocatalysis process. To exclude dye sensitization process, the colorless organic pollutants such as ciprofloxacin (CIP) antibiotic was selected for evaluating the photocatalytic activity of the WO3-Cu-1.0 hybrid. As shown in Fig. 5(b), it is hard to remove the ciprofloxacin antibiotic through direct photolysis or in the presence of pure WO3 product. However, when the WO3-Cu-1.0 was used as the photocatalyst, about 75% of the ciprofloxacin was removed in 4.5 h. As a matter of fact, the Congo Red cannot be degraded under the light irradiation for 4 h. This result indicated that photocatalysis occurred during the Congo Red removal process. Fig. 5(c) shows the ESR spectra of DMPO•OH signal of WO3-Cu-1.0 composite. It can be clearly observed the obvious characteristic peak of •OH for the WO3-Cu-1.0 composite upon light irradiation, which further confirms the reactive oxygen species during the Congo Red photodegradation.
Figure 5.(a) Effect of scavengers on the photocatalytic degradation and (b) photocatalytic degradation of CIP (40 mL, 20 mg/L) upon WO3-Cu-1.0 composite (20 mg); (c) DMPO •OH EPR spin-trapping spectra of WO3-Cu-1.0 composite upon sun light irradiation by a 500 W Xe lamp
The separation efficiency of the electron-hole pairs on pure WO3 and WO3-Cu-1.0 hybrid was further studied by photocurrent and EIS experiments. As shown in Fig. 6(a, b), compared to WO3 nanocube, the WO3-Cu-1.0 hybrid had smaller arc radius and superior photocurrent. It reflected that the WO3-Cu-1.0 hybrid exhibited rapid interfacial charge transfer and electron-hole separation efficiency, which contributed to its higher photocatalytic capability[
Figure 6.(a) Photocurrent response and (b) EIS of WO3 and WO3-Cu-1.0, and (c) proposed pathways for photocatalytic degradation of Congo Red
As shown in Fig. 6(c), under visible light illumination, the WO3 was excited directly, and electrons (eC-B) and holes (hV+B) were produced upon the WO3. Subsequently, eC-B reacted with the O2 molecules on the surface to yield reactive oxygen radicals (•O2-), while hV+B was directly oxidized to generate •OH. Finally, the CR dye was degraded by the reactive oxygen radicals and/or hV+B[
3 Conclusion
In summary, cube-like WO3-Cu hybrid was successfully fabricated by a facile room temperature method. The involving of Cu particle did not tailor the structure of WO3 nanocubes, but had impact on its photocatalytic activity. Among the WO3-Cu hybrid, WO3-Cu-1.0 showed the highest efficiency towards Congo Red photodegradation. During the photocatalysis process, the generated holes and the •OH were the main active species. Based on photocurrent and EIS measurement, it was concluded that the enhancement of photocatalytic capability was mainly attributable to the higher charge transfer and lower electron- hole recombination of the WO3-Cu hybrid.
References
[1] Q GUO, C ZHOU, Z MA et al. Fundamentals of TiO2 photocatalysis: concepts, mechanisms, and challenges. Advanced Materials, 1901997(2019).
[6] J ZHANG L, S LI, K LIU B et al. Highly efficient CdS/WO3 photocatalysts: Z-Scheme photocatalytic mechanism for their enhanced photocatalytic H2 evolution under visible light. ACS Catalysis, 4, 3724-3729(2014).
[7] W ZENG, T CAI, Y LIU et al. An artificial organic-inorganic Z- scheme photocatalyst WO3@Cu@PDI supramolecular with excellent visible light absorption and photocatalytic activity. Chemical Engineering Journal, 381, 122691(2020).
[8] Y LI, Z LIU, Z GUO et al. Efficient WO3 photoanode modified by Pt layer and plasmonic Ag for enhanced charge separation and transfer to promote photoelectrochemical performances. ACS Sustainable Chemistry & Engineering, 7, 12582-12590(2019).
[9] K SUN, Q LU, C MA et al. Pt modified ultrafine WO3 nanofibers: a combined first-principles and experimental study. Materials Letters, 236, 267-270(2019).
[10] H GONG, Y ZHANG, Y CAO et al. Pt@Cu2O/WO3 composite photocatalyst for enhanced photocatalytic water oxidation performance. Applied Catalysis B: Environmental, 237, 309-317(2018).
[13] J ZHU, M ZHANG, J XIONG et al. Electrostatically assembled construction of ternary TiO2-Cu@C hybrid with enhanced solar-to- hydrogen evolution employing amorphous carbon dots as electronic mediator. Chemical Engineering Journal, 375, 121902(2019).
[14] J ZHU, G CHENG, J XIONG et al. Recent advances in Cu-based cocatalysts toward solar-to-hydrogen evolution: categories and roles. Solar RRL, 3, 1900256(2019).
[15] MI MALDONADO, A LÓPEZ-MARTÍN, G COLÓN et al. Solar pilot plant scale hydrogen generation by irradiation of Cu/TiO2 composites in presence of sacrificial electron donors. Applied Catalysis B: Environmental, 229, 15-23(2018).
[16] H WANG, Y WANG, A XU et al. Facile synthesis of a novel WO3/Ag2MoO4 particles-on-plate staggered type II heterojunction with improved visible-light photocatalytic activity in removing environmental pollutants. RSC Advances, 9, 34804-34813(2019).
[18] MA LARA, C JARAMILLO-PÁEZ, JA NAVÍO et al. Coupling of WO3 with anatase TiO2 sample with high {001} facet exposition: effect on the photocatalytic properties. Catalysis Today, 328, 142-148(2019).
[19] Y WEI, G CHENG, J XIONG et al. Synergistic impact of cocatalysts and hole scavenger for promoted photocatalytic H2 evolution in mesoporous TiO2-NiS
[20] D MAJHI, K DAS, A MISHRA et al. One pot synthesis of CdS/BiOBr/Bi2O2CO3: a novel ternary double Z-scheme heterostructure photocatalyst for efficient degradation of atrazine. Applied Catalysis B: Environmental, 260, 118222(2020).
[21] G CHENG, Y WEI, J XIONG et al. Same titanium glycolate precursor but different products: successful synthesis of twinned anatase TiO2 nanocrystals with excellent solar photocatalytic hydrogen evolution capability. Inorganic Chemistry Frontiers, 4, 1319-1329(2017).
[22] C HU, X ZHANG, X LI et al. Au photosensitized TiO2 ultrathin nanosheets with {001} exposed facets. Chemistry - A European Journal, 20, 13557-13560(2014).
[23] J TIAN, P HAO, N WEI et al. 3D Bi2MoO6 nanosheet/TiO2 nanobelt heterostructure: enhanced photocatalytic activities and photoelectochemistry performance. ACS Catalysis, 5, 4530-4536(2015).
[25] X HU, H ZHAO, Y LIANG et al. Energy level mediation of (BiO)2CO3
[26] X WANG, T LI, R YU et al. Highly efficient TiO2 single-crystal photocatalyst with spatially separated Ag and F- bi-cocatalysts: orientation transfer of photogenerated charges and their rapid interfacial reaction. Journal of Materials Chemistry A, 4, 8682-8689(2016).
[27] J ZHOU, Y LI, L YU et al. Facile
[30] Y LIU, G ZHU, J GAO et al. Enhanced photocatalytic activity of Bi4Ti3O12 nanosheets by Fe 3+-doping and the addition of Au nanoparticles: photodegradation of phenol and bisphenol A. Applied Catalysis B: Environmental, 200, 72-82(2017).
[31] M DIAK, M KLEIN, T KLIMCZUK et al. Photoactivity of decahedral TiO2 loaded with bimetallic nanoparticles: degradation pathway of phenol-1-13C and hydroxyl radical formation. Applied Catalysis B: Environmental, 200, 56-71(2017).
[32] AE GIANNAKAS, M ANTONOPOULOU, C DAIKOPOULOS et al. Characterization and catalytic performance of B-doped, B-N co-doped and B-N-F tri-doped TiO2 towards simultaneous Cr(VI) reduction and benzoic acid oxidation. Applied Catalysis B: Environmental, 184, 44-54(2016).
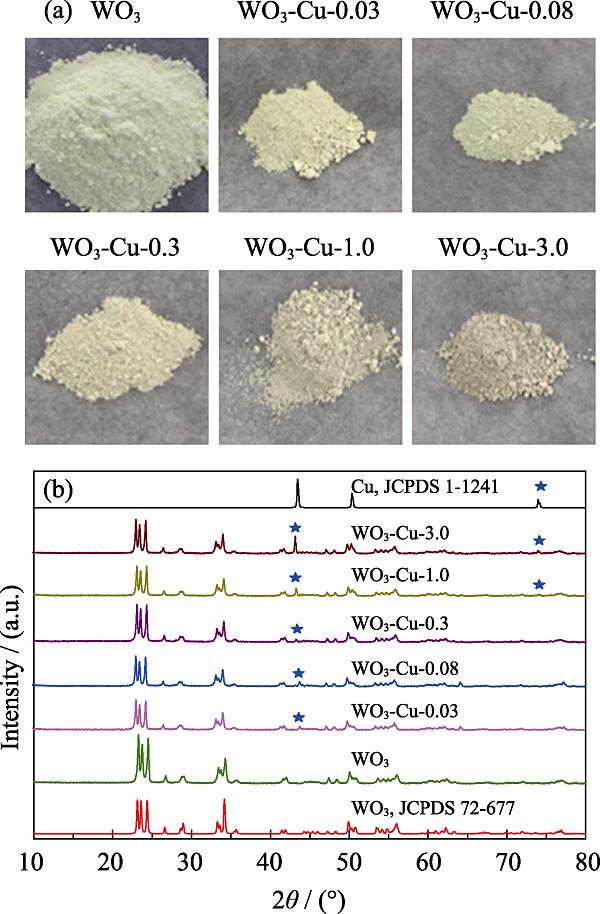
Set citation alerts for the article
Please enter your email address