
- Photonics Research
- Vol. 10, Issue 9, 2066 (2022)
Abstract
1. INTRODUCTION
Photonic quantum information processing has given birth to a wide array of emerging quantum technologies, such as quantum computing [1,2], quantum communications [3,4], quantum repeaters [5,6], and ultimately a full-fledged quantum Internet [7,8]. Implementing these techniques requires the bright and deterministic emission of background-free single photons into a given quantum state. Ideally, the single photons emitted at different time should be indistinguishable, including polarization, spatial mode, and transform-limited spectrotemporal profile, for high-visibility Hong–Ou–Mandel type quantum interference [9]. For on-chip quantum photonic circuits, single photons should be efficiently coupled to a waveguide for processing photons with high-fidelity operators on-chip [10].
So far, self-assembled semiconductor quantum dots (QDs), showing high quantum efficiency in solid-state quantum emitters, have become one of the most attractive candidates to serve as ideal single-photon emitters [11]. By eliminating dephasing and time jitter, pulsed resonant excitation on single QDs has achieved near-ideal single photons. Well-designed nano structures have shown the ability to improve the collection efficiency of emitted single photons and engineer their quantum properties by precisely controlling the environment of the emitter [12–14]. By combining resonant excitation with Purcell-enhanced microcavities [15–17], the generated near-ideal single-photon and entangled-photon pair sources have been efficiently extracted out of bulk.
In general, a cross-polarized excitation–collection scheme [18,19] is preferred for implementing pulsed resonant excitation of a QD. But this method inherently limits the collection efficiency of the generated single photons to
Sign up for Photonics Research TOC. Get the latest issue of Photonics Research delivered right to you!Sign up now
Integrating QDs within on-chip waveguide circuits is a promising approach to achieve high coupling efficiency and source scalability without a filter [24–26]. Here the coupling efficiency is defined as the number of single photons extracted from bulk and coupled into the fundamental mode of the on-chip waveguide per pumping pulse. The excitation and collection can be set orthogonally in planar nanophotonic waveguides, which can suppress the excitation laser efficiently even without any optical filter [27]. Suspended waveguides such as a photonic crystal waveguide [28] or nanobeam waveguide [29,30] are highly efficient platforms for integrated quantum circuits with single photons based on QDs, but have poor scalability due to their mechanical fragility. Moreover, for unpolarized quantum state of QDs, such as a charged exciton, nanobeam waveguides suffer from considerable loss due to other polarized single-photon emission, which is orthogonal to waveguide TE modes. Nanobeam waveguides rarely suppress the unwanted polarized single-photon emission; even in a photonic crystal waveguide, the suppression is not enough, retaining a Purcell factor
Here, we propose an elliptical Bragg resonator with coupling waveguides on a solid-state base to achieve near-unity polarization efficiency and coupling efficiency of 80% without post-filter operation. In addition, the proposed structures are placed on SiO2 buffer layers that can support large-scale implementation. The QD sits at the center about 400 nm away from the etching surface, avoiding the deterioration of QD fluorescence.
2. THEORETICAL SCHEME
To get an on-demand and truly scalable high-performance source of indistinguishable single photons, we propose an elliptical Bragg resonator with coupling waveguides on the SiO2 solid-state substrate [Fig. 1(a)]. This structure can achieve a background-free excitation signal without sacrificing system efficiency, while coupling the generated single photon into the planar waveguide fundamental mode with high efficiency.
Figure 1.Schematic diagram of elliptical Bragg resonator with coupling waveguides for generation of on-chip single photons. (a) The elliptical Bragg resonator with coupling waveguides consists of an elliptical disk with quantum emitter in the center, fully etched elliptical Bragg gratings, and coupling waveguides on the long axis of the elliptical disk. The substrate of the structure is a thick low-refractive-index layer (e.g., silica). (b) Cross section with superimposed cavity mode electric field of the elliptical Bragg resonator with coupling waveguides in
As shown in Fig. 1(a), a polarized microcavity called an elliptical Bragg resonator supports two-fold non-degenerate cavity modes [17], i.e., horizontal polarization (H-Pol) and vertical polarization (V-Pol) modes. The pulsed resonance excitation laser with H-Pol excites the solid-state quantum emitters (e.g., QDs) embedded in the central elliptical disk of the resonator. This excitation light, which cannot be coupled to the waveguide fundamental mode due to the space pattern mismatch and polarization orthogonality of their mode fields, will be suppressed efficiently in the on-chip waveguide. The elliptical Bragg resonator can also selectively enhance or suppress the different polarization fluorescence of the emitter in the middle of the resonator. Hence, only the excited emitter (e.g., QDs) matching the V-Pol resonating mode can emit V-Pol single-photon streams efficiently due to polarization-dependent Purcell enhancement [17]. These V-Pol single photons can be further coupled into the fundamental mode of the two waveguides with high coupling efficiency [see Fig. 1(b)]. There are some advantages of this scheme: (1) it can overcome the 50% efficiency limit of conventional resonance excitation and enhance the on-chip coupling efficiency of single photons; (2) the excitation light and single photons are distributed in different channels, which is essentially suitable for experimental implementation of the filter-free pulsed resonant excitation scheme; (3) it separates coherent single photons into two symmetric on-chip waveguides, functioning as an integration beam splitter, an essential component in photon-based quantum computing [23].
The on-chip coupling efficiency
3. ELLIPTICAL BRAGG RESONATORS
Circular Bragg resonators, tightly confining the light in a sub-
We design a GaAs-based elliptical Bragg resonator with the center disk embedding an InAs QD, surrounded by a first-order Bragg grating on the
The elliptical Bragg resonator supports two-fold orthogonal cavity modes, one in H-Pol and the other in V-Pol. These two modes are very sensitive to the size and ellipticity of the center disk. Here, the degree of ellipticity
When coupling a single-electron charged QD to this elliptical Bragg resonator, the inherent orthogonal polarization symmetry of QD emission will be broken. As a result, its spontaneous emission rate will be redistributed into the V-Pol and H-Pol. As shown in Fig. 2, a 1% ellipticity results in a 2.3 nm split between the Purcell factor peaks of the cavity H mode and cavity V mode [see Fig. 2(b)]. The splitting width will increase to 14 nm when increasing the ellipticity to 5% [see Fig. 2(c)]. The Purcell factor peak of the cavity H mode will blueshift out of our working wavelength window when the degree of ellipticity
Figure 2.Purcell factor distribution and polarization efficiency of the resonator with different ellipticities. Purcell factor as a function of wavelength with different ellipticities of (a)
Figure 2(e) shows the polarization efficiency of the elliptical Bragg resonator with different ellipticities. Here, the polarization efficiency
For the circular Bragg resonator [
4. COMBINATION ELLIPTICAL BRAGG RESONATORS WITH COUPLING WAVEGUIDES
After designing the elliptical Bragg resonator with near-unity polarization efficiency, we add the coupling waveguides to achieve both a high
As the waveguide approaches the central disk, more photons can leak out to the waveguide, achieving a high
As shown in Figs. 3(a) and 3(b), the
Figure 3.Elliptical Bragg resonator with coupling waveguide for coupling polarized single photons. The
5. RESULTS AND DISCUSSION
The single-photon performance and fabrication feasibility of the elliptical Bragg resonator with coupling waveguides will be discussed after setting the parameters of this device.
Figure 4.Robustness of the elliptical Bragg resonator with coupling waveguide on
6. CONCLUSION
In conclusion, we have proposed an elliptical Bragg resonator with coupling waveguides for deterministic pulsed resonant excitation of single photons. Our simulations show that up to 80% of single photons can be coupled into the on-chip waveguide with a Purcell factor of seven. Furthermore, an extinction ratio of
APPENDIX A: METHODS
The simulation is done by the finite-difference time-domain (FDTD) method from Lumerical Inc.
APPENDIX B: FIRST-ORDER AND SECOND-ORDER BRAGG GRATINGS
For light propagating along a Bragg grating, the Bragg condition can be expressed as follows:
The grating diffraction should satisfy the following relations:
For a first-order Bragg grating [Fig.
Figure 5.Diffraction of (a) first-order and (b) second-order Bragg gratings. The yellow bold arrow indicates the initial light propagating wave vector. Black arrows superimposed on grating are the corresponding reciprocal lattice vector
References
[1] P. Kok, W. J. Munro, K. Nemoto, T. C. Ralph, J. P. Dowling, G. J. Milburn. Linear optical quantum computing with photonic qubits. Rev. Mod. Phys., 79, 135-174(2007).
[2] H.-S. Zhong, H. Wang, Y.-H. Deng, M.-C. Chen, L.-C. Peng, Y.-H. Luo, J. Qin, D. Wu, X. Ding, Y. Hu, P. Hu, X.-Y. Yang, W.-J. Zhang, H. Li, Y. Li, X. Jiang, L. Gan, G. Yang, L. You, Z. Wang, L. Li, N.-L. Liu, C.-Y. Lu, J.-W. Pan. Quantum computational advantage using photons. Science, 370, 1460-1463(2020).
[3] Z. Qi, Y. Li, Y. Huang, J. Feng, Y. Zheng, X. Chen. A 15-user quantum secure direct communication network. Light Sci. Appl., 10, 183(2021).
[4] C. Schimpf, M. Reindl, D. Huber, B. Lehner, F. S. C. da Silva, S. Manna, M. Vyvlecka, P. Walther, A. Rastelli. Quantum cryptography with highly entangled photons from semiconductor quantum dots. Sci. Adv., 7, eabe8905(2021).
[5] K. Azuma, K. Tamaki, H.-K. Lo. All-photonic quantum repeaters. Nat. Commun., 6, 6787(2015).
[6] X. Liu, J. Hu, Z.-F. Li, X. Li, P.-Y. Li, P.-J. Liang, Z.-Q. Zhou, C.-F. Li, G.-C. Guo. Heralded entanglement distribution between two absorptive quantum memories. Nature, 594, 41-45(2021).
[7] S. Brito, A. Canabarro, R. Chaves, D. Cavalcanti. Statistical properties of the quantum internet. Phys. Rev. Lett., 124, 210501(2020).
[8] S. Wehner, D. Elkouss, R. Hanson. Quantum internet: a vision for the road ahead. Science, 362, eaam9288(2018).
[9] H. Ollivier, S. E. Thomas, S. C. Wein, I. M. de Buy Wenniger, N. Coste, J. C. Loredo, N. Somaschi, A. Harouri, A. Lemaitre, I. Sagnes, L. Lanco, C. Simon, C. Anton, O. Krebs, P. Senellart. Hong-Ou-Mandel interference with imperfect single photon sources. Phys. Rev. Lett., 126, 063602(2021).
[10] S. Hepp, M. Jetter, S. L. Portalupi, P. Michler. Semiconductor quantum dots for integrated quantum photonics. Adv. Quantum Technol., 2, 1900020(2019).
[11] P. Senellart, G. Solomon, A. White. High-performance semiconductor quantum-dot single-photon sources. Nat. Nanotechnol., 12, 1026-1039(2017).
[12] B. Chen, Y. Wei, T. Zhao, S. Liu, R. Su, B. Yao, Y. Yu, J. Liu, X. Wang. Bright solid-state sources for single photons with orbital angular momentum. Nat. Nanotechnol., 16, 302-307(2021).
[13] Ł. Dusanowski, D. Köck, E. Shin, S.-H. Kwon, C. Schneider, S. Höfling. Purcell-enhanced and indistinguishable single-photon generation from quantum dots coupled to on-chip integrated ring resonators. Nano Lett., 20, 6357-6363(2020).
[14] J. Liu, R. Su, Y. Wei, B. Yao, S. F. C. da Silva, Y. Yu, J. Iles-Smith, K. Srinivasan, A. Rastelli, J. Li, X. Wang. A solid-state source of strongly entangled photon pairs with high brightness and indistinguishability. Nat. Nanotechnol., 14, 586-593(2019).
[15] H. Ollivier, I. Maillette de Buy Wenniger, S. Thomas, S. C. Wein, A. Harouri, G. Coppola, P. Hilaire, C. Millet, A. Lemaître, I. Sagnes, O. Krebs, L. Lanco, J. C. Loredo, C. Antón, N. Somaschi, P. Senellart. Reproducibility of high-performance quantum dot single-photon sources. ACS Photon., 7, 1050-1059(2020).
[16] N. Tomm, A. Javadi, N. O. Antoniadis, D. Najer, M. C. Löbl, A. R. Korsch, R. Schott, S. R. Valentin, A. D. Wieck, A. Ludwig, R. J. Warburton. A bright and fast source of coherent single photons. Nat. Nanotechnol., 16, 399-403(2021).
[17] H. Wang, Y.-M. He, T. H. Chung, H. Hu, Y. Yu, S. Chen, X. Ding, M. C. Chen, J. Qin, X. Yang, R.-Z. Liu, Z. C. Duan, J. P. Li, S. Gerhardt, K. Winkler, J. Jurkat, L.-J. Wang, N. Gregersen, Y.-H. Huo, Q. Dai, S. Yu, S. Höfling, C.-Y. Lu, J.-W. Pan. Towards optimal single-photon sources from polarized microcavities. Nat. Photonics, 13, 770-775(2019).
[18] A. V. Kuhlmann, J. Houel, D. Brunner, A. Ludwig, D. Reuter, A. D. Wieck, R. J. Warburton. A dark-field microscope for background-free detection of resonance fluorescence from single semiconductor quantum dots operating in a set-and-forget mode. Rev. Sci. Instrum., 84, 073905(2013).
[19] F. Liu, A. J. Brash, J. O’Hara, L. M. P. P. Martins, C. L. Phillips, R. J. Coles, B. Royall, E. Clarke, C. Bentham, N. Prtljaga, I. E. Itskevich, L. R. Wilson, M. S. Skolnick, A. M. Fox. High Purcell factor generation of indistinguishable on-chip single photons. Nat. Nanotechnol., 13, 835-840(2018).
[20] U. M. Gür, M. Mattes, S. Arslanagić, N. Gregersen. Elliptical micropillar cavity design for highly efficient polarized emission of single photons. Appl. Phys. Lett., 118, 061101(2021).
[21] M. Varnava, D. E. Browne, T. Rudolph. How good must single photon sources and detectors be for efficient linear optical quantum computation?. Phys. Rev. Lett., 100, 060502(2008).
[22] J. C. Loredo, M. A. Broome, P. Hilaire, O. Gazzano, I. Sagnes, A. Lemaitre, M. P. Almeida, P. Senellart, A. G. White. Boson sampling with single-photon Fock states from a bright solid-state source. Phys. Rev. Lett., 118, 130503(2017).
[23] H. Wang, J. Qin, X. Ding, M.-C. Chen, S. Chen, X. You, Y.-M. He, X. Jiang, L. You, Z. Wang, C. Schneider, J. J. Renema, S. Höfling, C.-Y. Lu, J.-W. Pan. Boson sampling with 20 input photons and a 60-mode interferometer in a 1014-dimensional Hilbert space. Phys. Rev. Lett., 123, 250503(2019).
[24] M. Arcari, I. Söllner, A. Javadi, S. Lindskov Hansen, S. Mahmoodian, J. Liu, H. Thyrrestrup, E. H. Lee, J. D. Song, S. Stobbe, P. Lodahl. Near-unity coupling efficiency of a quantum emitter to a photonic crystal waveguide. Phys. Rev. Lett., 113, 093603(2014).
[25] J. Q. Grim, A. S. Bracker, M. Zalalutdinov, S. G. Carter, A. C. Kozen, M. Kim, C. S. Kim, J. T. Mlack, M. Yakes, B. Lee, D. Gammon. Scalable in operando strain tuning in nanophotonic waveguides enabling three-quantum-dot superradiance. Nat. Mater., 18, 963-969(2019).
[26] R. Uppu, H. T. Eriksen, H. Thyrrestrup, A. D. Uğurlu, Y. Wang, S. Scholz, A. D. Wieck, A. Ludwig, M. C. Löbl, R. J. Warburton, P. Lodahl, L. Midolo. On-chip deterministic operation of quantum dots in dual-mode waveguides for a plug-and-play single-photon source. Nat. Commun., 11, 3782(2020).
[27] H. Thyrrestrup, G. Kiršanskė, H. Le Jeannic, T. Pregnolato, L. Zhai, L. Raahauge, L. Midolo, N. Rotenberg, A. Javadi, R. Schott, A. D. Wieck, A. Ludwig, M. C. Löbl, I. Söllner, R. J. Warburton, P. Lodahl. Quantum optics with near-lifetime-limited quantum-dot transitions in a nanophotonic waveguide. Nano Lett., 18, 1801-1806(2018).
[28] R. Uppu, F. T. Pedersen, Y. Wang, C. T. Olesen, C. Papon, X. Zhou, L. Midolo, S. Scholz, A. D. Wieck, A. Ludwig, P. Lodahl. Scalable integrated single-photon source. Sci. Adv., 6, eabc8268(2020).
[29] M. N. Makhonin, J. E. Dixon, R. J. Coles, B. Royall, I. J. Luxmoore, E. Clarke, M. Hugues, M. S. Skolnick, A. M. Fox. Waveguide coupled resonance fluorescence from on-chip quantum emitter. Nano Lett., 14, 6997-7002(2014).
[30] G. Reithmaier, M. Kaniber, F. Flassig, S. Lichtmannecker, K. Müller, A. Andrejew, J. Vučković, R. Gross, J. J. Finley. On-chip generation, routing, and detection of resonance fluorescence. Nano Lett., 15, 5208-5213(2015).
[31] P. Stepanov, A. Delga, X. Zang, J. Bleuse, E. Dupuy, E. Peinke, P. Lalanne, J.-M. Gérard, J. Claudon. Quantum dot spontaneous emission control in a ridge waveguide. Appl. Phys. Lett., 106, 041112(2015).
[32] S. Kalliakos, Y. Brody, A. J. Bennett, D. J. P. Ellis, J. Skiba-Szymanska, I. Farrer, J. P. Griffiths, D. A. Ritchie, A. J. Shields. Enhanced indistinguishability of in-plane single photons by resonance fluorescence on an integrated quantum dot. Appl. Phys. Lett., 109, 151112(2016).
[33] J. Liu, K. Konthasinghe, M. Davanço, J. Lawall, V. Anant, V. Verma, R. Mirin, S. W. Nam, J. D. Song, B. Ma, Z. S. Chen, H. Q. Ni, Z. C. Niu, K. Srinivasan. Single self-assembled InAs/GaAs quantum dots in photonic nanostructures: the role of nanofabrication. Phys. Rev. Appl., 9, 064019(2018).
[34] C. L. Dreeßen, C. Ouellet-Plamondon, P. Tighineanu, X. Zhou, L. Midolo, A. S. Sørensen, P. Lodahl. Suppressing phonon decoherence of high performance single-photon sources in nanophotonic waveguides. Quantum Sci. Technol., 4, 015003(2018).
[35] D. Najer, I. Söllner, P. Sekatski, V. Dolique, M. C. Löbl, D. Riedel, R. Schott, S. Starosielec, S. R. Valentin, A. D. Wieck, N. Sangouard, A. Ludwig, R. J. Warburton. A gated quantum dot strongly coupled to an optical microcavity. Nature, 575, 622-627(2019).
[36] M. Davanço, M. T. Rakher, D. Schuh, A. Badolato, K. Srinivasan. A circular dielectric grating for vertical extraction of single quantum dot emission. Appl. Phys. Lett., 99, 041102(2011).
[37] L. Li, E. H. Chen, J. Zheng, S. L. Mouradian, F. Dolde, T. Schröder, S. Karaveli, M. L. Markham, D. J. Twitchen, D. Englund. Efficient photon collection from a nitrogen vacancy center in a circular Bullseye grating. Nano Lett., 15, 1493-1497(2015).
[38] B. Yao, R. Su, Y. Wei, Z. Liu, T. Zhao, J. Liu. Design for hybrid circular Bragg gratings for a highly efficient quantum-dot single-photon source. J. Korean Phys. Soc., 73, 1502-1505(2018).
[39] L. Rickert, T. Kupko, S. Rodt, S. Reitzenstein, T. Heindel. Optimized designs for telecom-wavelength quantum light sources based on hybrid circular Bragg gratings. Opt. Express, 27, 36824-36837(2019).
[40] S. Kolatschek, C. Nawrath, S. Bauer, J. Huang, J. Fischer, R. Sittig, M. Jetter, S. L. Portalupi, P. Michler. Bright Purcell enhanced single-photon source in the telecom O-band based on a quantum dot in a circular Bragg grating. Nano Lett., 21, 7740-7745(2021).
[41] R. Harper, S. T. Flammia, J. J. Wallman. Efficient learning of quantum noise. Nat. Phys., 16, 1184-1188(2020).
[42] S. Kako, C. Santori, K. Hoshino, S. Götzinger, Y. Yamamoto, Y. Arakawa. A gallium nitride single-photon source operating at 200 K. Nat. Mater., 5, 887-892(2006).
[43] J. Liu, M. I. Davanço, L. Sapienza, K. Konthasinghe, J. V. de Miranda Cardoso, J. D. Song, A. Badolato, K. Srinivasan. Cryogenic photoluminescence imaging system for nanoscale positioning of single quantum emitters. Rev. Sci. Instrum., 88, 023116(2017).
[44] C. Antón, J. C. Loredo, G. Coppola, H. Ollivier, N. Viggianiello, A. Harouri, N. Somaschi, A. Crespi, I. Sagnes, A. Lemaître, L. Lanco, R. Osellame, F. Sciarrino, P. Senellart. Interfacing scalable photonic platforms: solid-state based multi-photon interference in a reconfigurable glass chip. Optica, 6, 1471-1477(2019).
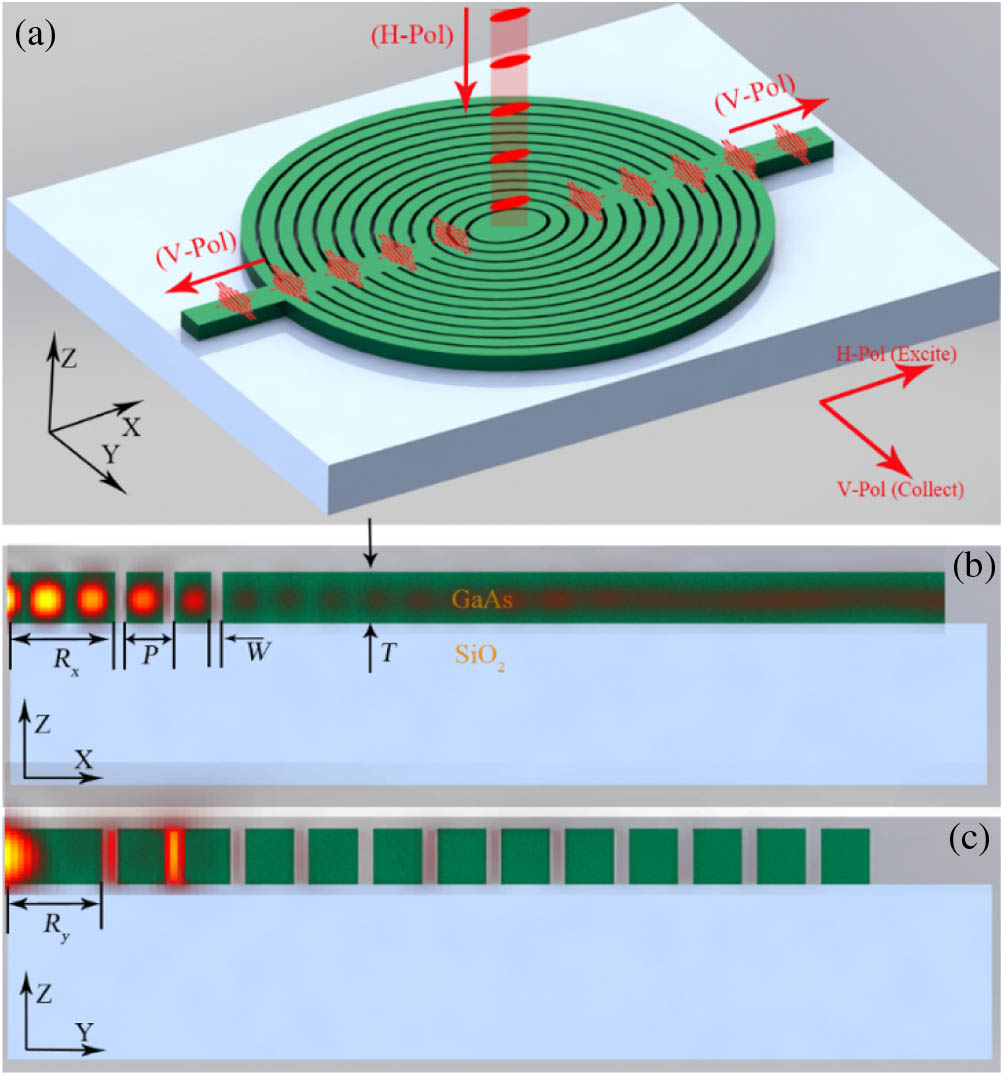
Set citation alerts for the article
Please enter your email address