T.-T. Qin, W. Luo, H.-Y. Lan, W.-M. Wang. Ultrafast probing of plasma ion temperature in proton–boron fusion by nuclear resonance fluorescence emission spectroscopy[J]. Matter and Radiation at Extremes, 2022, 7(3): 035901

Search by keywords or author
- Matter and Radiation at Extremes
- Vol. 7, Issue 3, 035901 (2022)
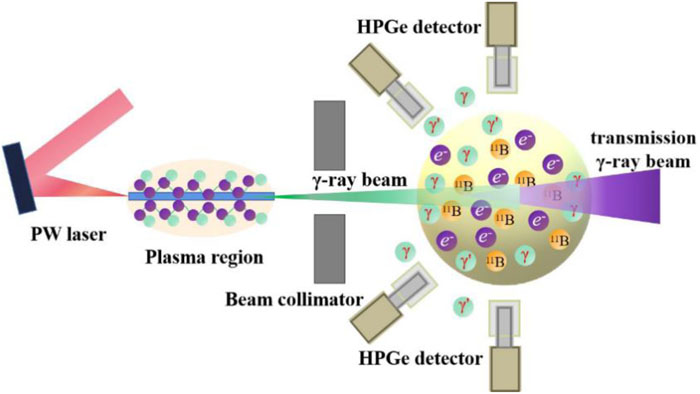
Fig. 1. Schematic of ultrafast probing of ion temperature in a hot 11B plasma by Doppler broadening of NRF. An intense γ-ray beam can be efficiently generated from submicrometer wires irradiated by a recently available PW laser. After collimation, the γ-ray beam is fired into a 11B pellet, which is located 11 cm downstream from the wire position and has a small radius of 2 mm, whereupon NRF interactions are excited. This is followed by emission of the characteristic photons. Owing to Doppler broadening, the NRF emission spectra contain information about ion temperature. To record these NRF signals efficiently, 24 high-purity germanium (HPGe) detectors are distributed in two concentric rings and are located at 90° and 135° with respect to the incident γ beam. The distance between the detectors and the pellet is 30 cm. For each detector, the germanium crystal used is 8.6 cm thick and has radius 4.2 cm. In our case, owing to the small size of the 11B pellet, a large number of energetic γ rays will penetrate through the pellet, forming a transmitted γ-ray beam. For better visualization, the figure is not to scale.
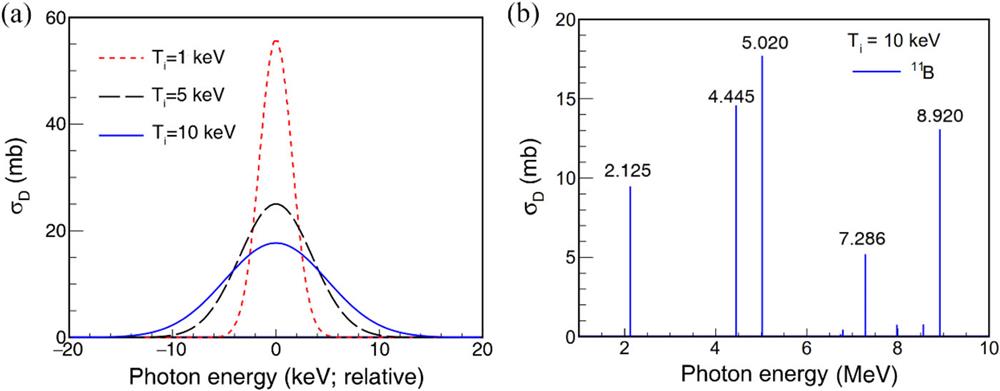
Fig. 2. (a) Dependence of Doppler-broadened cross section on 11B ion temperature at E r = 5.020 MeV. (b) Doppler-broadened cross section of 11B as a function of photon energy.
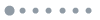
Fig. 3. Spectral–angular distribution of γ-ray pulses emitted from laser-illuminated wires under laser powers P 0 = 0.5 PW (a), 1.0 PW (b), 2.5 PW (c), and 5.0 PW (d).
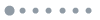
Fig. 4. Spectra of the γ-ray beams with collimation angle <1° at four different laser peak powers. The tails of these spectra are overlaid with exponential temperature fits, shown by broken lines.
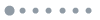
Fig. 5. Simulation results for a collimated intense γ-ray beam with effective temperature T γ = 70 MeV at a laser power of 2.5 PW. (a) γ-ray intensity recorded by 24 HPGe detectors as a function of observing angle θ and energy (which is represented by the radius in the polar figure). (b) γ-ray spectrum showing five NRF signatures and a few escape peaks. The inset shows an enlarged view of one NRF signature at 5.020 MeV. The dashed line is a fitting curve of a Gaussian distribution.
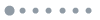
Fig. 6. (a) Fitting curves of NRF peaks at 5.020 MeV. A collimated intense γ-ray beam with temperature T γ = 70 MeV at laser power 2.5 PW is used for the simulation. (b) Values of δE sim, δE , 2.355Δ, and δE D at 5.020 MeV as functions of ion temperature T i .
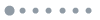
Fig. 7. Dependence of the detected NRF yield (a) and areal density (b) on laser peak power with 11B ions at resonance energies of 2.125, 4.445, 5.020, 7.286, and 8.920 MeV. The NRF yield is fixed at 100 for calculating the required areal density in (b).
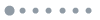
Fig. 8. Threshold areal density required for four aneutronic fusion materials. The resonance energy E r is selected to be 3.563, 0.478, 5.020, and 6.324 MeV for 6Li, 7Li, 11B, and 15N, respectively. A collimated intense γ-ray beam with temperature T γ = 38 MeV at 1.0 PW laser power is used.
|
Table 1. Integrated NRF cross sections Iσ for principal transitions 0 → r → 0 in 6,7Li, 11B, and 15N at their characteristic energies Er and the estimated thresholds to probe ion temperature under the premise that δEsim ≥ 2δED.
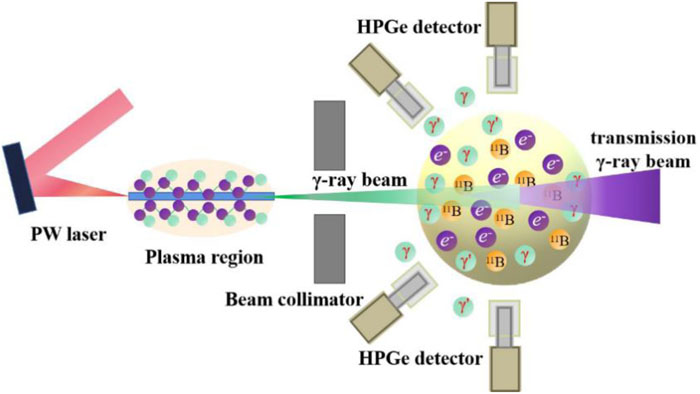
Set citation alerts for the article
Please enter your email address