Author Affiliations
1Laser & Fiber Electronics Group, Faculty of Electronics, Wrocław University of Science and Technology, Wybrzeze Wyspianskiego 27, 50-370 Wroclaw, Poland2Department of Optics and Photonics, Faculty of Fundamental Problems of Technology, Wroclaw University of Science and Technology, Wybrzeze Wyspianskiego 27, 50-370 Wroclaw, Poland3Laboratory of Optical Fiber Technology, Maria Curie-Sklodowska University, pl. M. Curie-Sklodowskiej 3, Lublin, Polandshow less
Abstract
We report generation of sub-100 fs pulses tunable from 1700 to 2100 nm via Raman soliton self-frequency shift. The nonlinear shift occurs in a highly nonlinear fiber, which is pumped by an Er-doped fiber laser. The whole system is fully fiberized, without the use of any free-space optics. Thanks to its exceptional simplicity, the setup can be considered as an alternative to mode-locked Tm- and Ho-doped fiber lasers.1. INTRODUCTION
Ultrashort-pulse fiber lasers operating in the 1.9–2.1 μm are currently one of the most intensively investigated branches of laser technology [1]. The main interest in such sources is driven by a great number of their applications. Efficient and compact 2 μm sources are widely used, e.g., for mid-infrared supercontinuum (SC) generation in photonic crystal fibers [2] and pumping of optical parametric oscillators [3,4] as well as in medical procedures [5,6]. Most commonly, 2 μm femtosecond pulses are generated from mode-locked fiber lasers based on Tm- or Ho-doped gain fibers, utilizing various mode-locking techniques, e.g., nonlinear polarization evolution (NPE) [7–9], graphene [10,11], or carbon nanotube saturable absorbers [12]. The simplest fiber lasers, namely, soliton lasers with all-anomalous dispersion cavities, are capable of generating sub-600 fs pulses at 2 μm to date [10,11]. Generation of shorter pulses requires careful dispersion management of the cavity and external compression of the pulses. As an example, sub-100 fs pulse generation at 2.06 μm from a Ho-doped fiber laser was demonstrated by Li et al. [7]. The authors in Ref. [8] obtained 130 fs pulses from a Tm-doped fiber laser. However, dispersion management makes the fiber laser setups much more complex than soliton oscillators. Additionally, the most impressive performance was achieved from lasers utilizing NPE mode-locking mechanism, which requires free-space bulk optics [7–9,13]. This makes the experimental setups vulnerable to external disturbances and limits their practical field applications. Dispersion-managed 2 μm lasers also can be developed in all-fiber configuration, with pulse compression performed in standard single mode fibers. However, the pulses are usually much longer than the transform limit [12,14].
An efficient alternative to conventional Tm- and Ho-doped fiber lasers is based on generating the 2 μm radiation utilizing the Raman soliton self-frequency shift (SSFS) effect occurring in highly nonlinear fibers (HNLFs) [15]. This approach allows to generate 4.3 μm radiation with the use of fluoride fibers and high-energy laser source [16]. This effect also can be used for, e.g., generation of carrier-envelope offset-free radiation at 1.56 μm, as demonstrated by Krauss et al. [17]. The concept of using compact 1.56 μm lasers and shifting it via SSFS toward 2 μm utilizing long sections of standard fibers was presented by Nishizawa and Goto [18]. This wavelength range also can be obtained by using SSFS process in suspended core tellurite fiber [19] and -doped silica fibers [20]. However, the previous works report generation of multi-order solitons, which significantly impede appropriate filtering and reduce the shifted soliton energy.
Here, we report generation of sub-100 fs pulses tunable from 1700 to 2100 nm via SFSS effect in HNLF pumped by a compact Er-doped fiber laser (EDFL). The total number of components in the setup is extremely minimized, in order to achieve simplicity, robustness, and cost-effectiveness. The average output power of the shifted solitons reaches 20 mW (at 2100 nm), with 45 MHz repetition rate. We have observed only the first order of Raman soliton generation in the entire tuning range (confirmed by numerical simulations), which increases the practical applicability of the presented setup.
Sign up for Photonics Research TOC. Get the latest issue of Photonics Research delivered right to you!Sign up now
2. EXPERIMENTAL SETUP
The experimental setup used for generation of the frequency-shifted pulses is depicted in Fig. 1. As a source of seed pulses, a simple graphene-based soliton EDFL followed by a bi-directionally pumped Er-doped fiber amplifier (EDFA) was used. The oscillator in combination with the EDFA delivers pulses as short as 27 fs with 115 mW of average power at 45.5 MHz repetition rate. In our experiment, the output power of the EDFA was tuned just by changing the power of the co-directional pump laser (Pump 1). In this case, any change in the pump power also influences the duration of the 1560 nm pulse. Therefore, the shortest 27 fs pulse is obtained only at the maximum available pump power; at lower pump powers, the output pulse broadens up to 300 fs. The pulse durations measured at different output powers are shown in Table 1 (first two columns). Details on the seed design can be found in one of our previous papers [21].
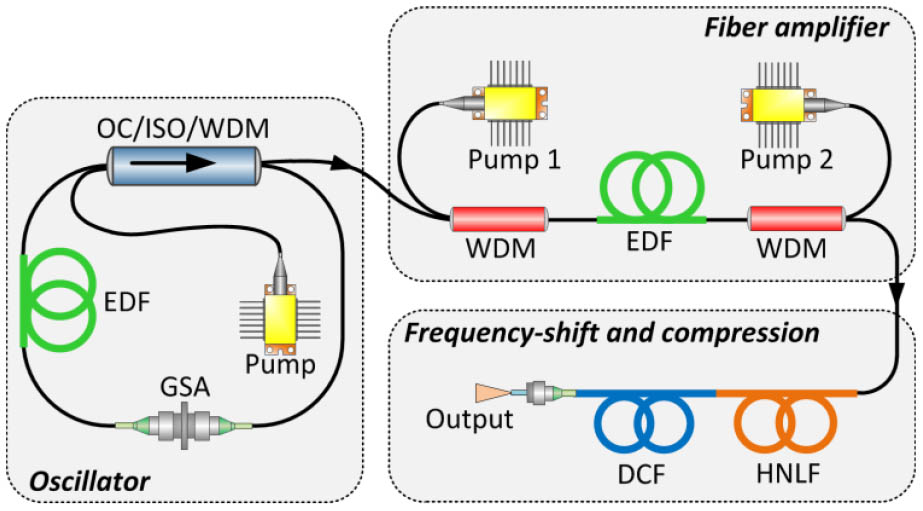
Figure 1.Experimental setup of the all-fiber frequency-shifted laser. EDF: Erbium-doped fiber; GSA: graphene saturable absorber; WDM: wavelength division multiplexer; OC/ISO/WDM—output coupler/isolator/WDM hybrid component.
Pin(mW) | τin(fs) | λSSFS(nm) | ΔλSSFS(nm) | PSSFS(mW) | LDCF(cm) | τSSFS(fs) |
39 | 300 | 1700 | 64 | — | 49 | 72 |
46 | 267 | 1750 | 72 | — | 59 | 68 |
53 | 236 | 1800 | 68 | 9.8 | 76 | 76 |
60 | 206 | 1850 | 64 | 12.4 | 76 | 77 |
72 | 145 | 1900 | 71 | 13.3 | 88 | 80 |
79 | 118 | 1950 | 85 | 16.1 | 115 | 83 |
85 | 76 | 2000 | 80 | 16.4 | 148 | 88 |
101 | 30 | 2050 | 101 | 19.5 | 178 | 92 |
115 | 27 | 2100 | 86 | 19.8 | 210 | 107 |
Table 1. Summary of the Obtained Results
The SSFS occurs in an in-house-developed photonic crystal HNLF. Afterward, the frequency-shifted solitons are spectrally broadened in a segment of a dispersion-compensating fiber (DCF, model DCF-2000 from OFS Labs). It is worth emphasizing that the whole system is entirely fiberized, without using any free-space bulk optics. The oscillator and the amplifier are made of polarization maintaining (PM) fibers and components, which ensure long-term stability and invulnerability to any external disturbances. All fibers in the setup (even the DCF and HNLF) were spliced using an arc-fusion splicer (Fujikura FSM-100P).
The silica HNLF used in the experiment was fabricated in the Laboratory of Optical Fiber Technology, Maria Curie–Sklodowska University, Lublin, Poland. The fiber preform was stacked from silica rods (cladding), and the germanium-doped rod fabricated by modified chemical vapor deposition method (core). The doping level was 18 mol%. Based on the scanning electron microscope (SEM) image of the fiber end facet (see Fig. 2) geometrical parameters of the microstructure were estimated and are as follows: germanium-doped core diameter ; diameter of the first air holes ring ; average diameter of the air holes in the first ring .
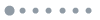
Figure 2.SEM images of the fabricated HNLF.
The dispersion profiles (Fig. 3) of both HNLF and DCF were measured by a white-light interferometric technique, employing a dispersion-balanced Mach–Zehnder interferometer [22] and a 1.1–2.0 μm SC source (NKT Photonics SuperK Versa), while spectral interferograms were registered using optical spectrum analyzers (OSA, Yokogawa AQ6375 and AQ6370B). The chromatic dispersion of the HNLF reaches zero at 1.25 μm and monotonically increases approaching at 2 μm. The propagation constants in the HNLF were determined using a Comsol Multiphysics Wave Optics Module, taking into account the actual fiber geometry (based on the SEM image, Fig. 2) and the material dispersion of pure and doped silica glass. The calculated effective mode area () reaches at 1560 nm wavelength, which results in an effective nonlinear coefficient of , while at 2000 nm equals to , and , for nonlinear refractive index .
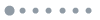
Figure 3.Measured dispersion characteristics of the HNLF and DCF fibers (dotted lines). Blue solid line represents the HNLF dispersion curve calculated based on the actual geometry of the fiber. Red solid line is the polynomial fitting of the measured DCF-2000 dispersion curve.
3. EXPERIMENTAL RESULTS
The performance of the laser was characterized using the following equipment: optical spectrum—Yokogawa AQ6375 OSA, pulse duration—Femtochrome FR-103XL second harmonic autocorrelator with 1000 nm photomultiplier, power—Gentec Maestro power meter with XLP12-3S-VP detector.
In our measurements, we have recorded the following parameters of the laser system: central wavelength of the shifted soliton (); its 3 dB bandwidth (); average output power of the filtered soliton (); its pulse duration (). The parameters were measured at certain conditions, i.e., at defined 1.56 μm signal input power (), input pulse duration (), and the length of the compressing fiber (). The obtained results are summarized in Table 1. The SSFS spectra measured at different input power levels are depicted in Fig. 4. We observed a soliton shift up to 2100 nm, which is easily tunable only by changing the power of the 1560 nm signal. The experimental data are supported by numerical calculations (dashed red line in Fig. 4). We adapted a MATLAB code for solving the numerically generalized nonlinear Schrödinger equation, developed by Travers et al. [23]. In our calculations, we accounted full measured group velocity dispersion curves and introduced a correction to account wavelength-dependent effective mode areas (based on finite element method calculations) [24]. Good agreement between the theoretical calculations and experimental results was achieved.
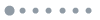
Figure 4.Measured (blue line) and calculated (red dots) optical spectra at the DCF output for different pumping powers. Spectra between 1800–1920 nm are affected by water absorption lines.
The dependence of the average output power of the soliton versus its central wavelength is presented in Fig. 5. For this measurement, the shifted solitons were filtered out from the entire spectrum using a bandpass filter centered at 2000 nm and with 500 nm FWHM (Thorlabs FB2000-500), which means that the power levels are unaffected by the residual pump. At highest frequency shifts (2050–2100 nm), the average power of the soliton almost reaches 20 mW. The ratio of the pump power and the shifted soliton power is at the level of 5:1, which is comparable with previous reports on SSFS lasers [25], and remains constant over the whole tuning range. The power of the 1700–1750 nm solitons was not measured due to lack of a proper bandpass filter.
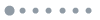
Figure 5.Average output power of the frequency-shifted soliton (filtered from the entire spectrum) at different central wavelengths.
Figure 6 depicts the obtained autocorrelation traces of the shifted solitons at different wavelengths. Due to the dispersion slope of the used DCF, it was necessary to adjust the DCF length at each wavelength in order to obtain the shortest pulse duration. We were able to achieve pulses shorter than 100 fs in the entire tuning range of our system (except the 2100 nm soliton with 107 fs). The solitons at wavelengths shorter than 1700 nm could not be correctly measured because they exceeded the wavelength range of the available bandpass filter (1750–2250 nm). When measured without any filter, the autocorrelation signal was strongly affected by the 1560 nm residual pump, due to small temporal separation between the pump and shifted soliton at the output of the fiber. For shifts higher than 1700 nm, the soliton is well-separated from the pump and can be easily measured with the autocorrelator. It is worth mentioning that the DCF in our setup broadens the output spectrum and enables shortening of the soliton. The pulse duration measured directly after the HNLF varies between 140 to 200 fs. We found that, by splicing the DCF to the HNLF, we might increase the FWHM of the spectrum even by a factor of 3. This gives the possibility to further compress the pulses to shorter durations. Figure 7 depicts a comparison between the soliton shifted to 2050 nm (the broadest spectrum observed in our study) taken directly from the HNLF output and after spectral broadening and temporal compression in the DCF. It can be seen that the duration shortens roughly by a factor of 2. The pulses are, however, longer than the transform limit. This suggests that the pulses might be further compressed with, e.g., a prism-based compressor; nevertheless, shorter duration could not be achieved with the use of DCF.
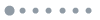
Figure 6.Measured pulse autocorrelations of the frequency-shifted solitons at different central wavelengths from 1700 to 2100 nm.
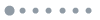
Figure 7.Comparison between the (a) optical spectrum and (b) pulse duration of the soliton centered at 2050 nm before and after the DCF-2000 fiber.
4. SUMMARY AND CONCLUSIONS
Summarizing, we have demonstrated a simple, fully fiberized soliton frequency shifting experiment. The SSFS occurs in an in-house-developed highly nonlinear photonic crystal fiber, which is pumped by a graphene-based Er-doped fiber oscillator. The presented setup is capable of generating sub-100 fs pulses in the range of 1700–2100 nm with average powers between 10–20 mW.
Our study shows that a simple frequency-shifting scheme applied to an EDFL allows us to generate sub-100 fs pulses in the 2 μm band with mW-level average powers. Therefore, such solution is an efficient alternative to Tm- or Ho-doped fiber lasers. Our setup outperforms in terms of pulse duration the previously reported dispersion-managed Tm- and Ho-doped fiber lasers [8–10,12]. Because Er-doped fibers and 1.55 μm components are significantly cheaper than Tm-doped fibers and 2 μm components, the SSFS scheme is, in our opinion, an excellent candidate for robust and cost-effective generation of ultrashort pulses in the 2 μm region.
References
[1] C. W. Rudy, M. J. F. Digonnet, R. L. Byer. Advances in 2-μm Tm-doped mode-locked fiber lasers. Opt. Fiber Technol., 20, 642-649(2014).
[2] M. Klimczak, B. Siwicki, B. Zhou, M. Bache, D. Pysz, O. Bang, R. Buczyński. Coherent supercontinuum bandwidth limitations under femtosecond pumping at 2 μm in all-solid soft glass photonic crystal fibers. Opt. Express, 24, 29406-29416(2016).
[3] A. Khodabakhsh, V. Ramaiah-Badarla, L. Rutkowski, A. C. Johansson, K. F. Lee, J. Jiang, C. Mohr, M. E. Fermann, A. Foltynowicz. Fourier transform and Vernier spectroscopy using an optical frequency comb at 3–5.4 μm. Opt. Lett., 41, 2541-2544(2016).
[4] N. Leindecker, A. Marandi, R. L. Byer, K. L. Vodopyanov, J. Jiang, I. Hartl, M. Fermann, P. G. Schunemann. Octave-spanning ultrafast OPO with 2.6–6.1 μm instantaneous bandwidth pumped by femtosecond Tm-fiber laser. Opt. Express, 20, 7046-7053(2012).
[5] B. Pal, K. Scholle, S. Lamrini, P. Koopmann, P. Fuhrberg. 2 μm laser sources and their possible applications. Frontiers in Guided Wave Optics and Optoelectronics(2010).
[6] N. M. Fried, K. E. Murray. High-power thulium fiber laser ablation of urinary tissues at 1.94 μm. J. Endourol., 19, 25-31(2005).
[7] P. Li, A. Ruehl, U. Grosse-Wortmann, I. Hartl. Sub-100 fs passively mode-locked holmium-doped fiber oscillator operating at 2.06 μm. Opt. Lett., 39, 6859-6862(2014).
[8] Y. Tang, A. Chong, F. W. Wise. Generation of 8 nJ pulses from a normal-dispersion thulium fiber laser. Opt. Lett., 40, 2361-2364(2015).
[9] F. Haxsen, A. Ruehl, M. Engelbrecht, D. Wandt, U. Morgner, D. Kracht. Stretched-pulse operation of a thulium-doped fiber laser. Opt. Express, 16, 20471-20476(2008).
[10] G. Sobon, J. Sotor, I. Pasternak, A. Krajewska, W. Strupinski, K. M. Abramski. All-polarization maintaining, graphene-based femtosecond Tm-doped all-fiber laser. Opt. Express, 23, 9339-9346(2015).
[11] J. Sotor, M. Pawliszewska, G. Sobon, P. Kaczmarek, A. Przewolka, I. Pasternak, J. Cajzl, P. Peterka, P. Honzátko, I. Kašík, W. Strupinski, K. Abramski. All-fiber Ho-doped mode-locked oscillator based on a graphene saturable absorber. Opt. Lett., 41, 2592-2595(2016).
[12] J. Wang, X. Liang, G. Hu, Z. Zheng, S. Lin, D. Ouyang, X. Wu, P. Yan, S. Ruan, Z. Sun, T. Hasan. 152 fs nanotube-mode-locked thulium-doped all-fiber laser. Sci. Rep., 6, 28885(2016).
[13] M. Engelbrecht, F. Haxsen, A. Ruehl, D. Wandt, D. Kracht. Ultrafast thulium-doped fiber-oscillator with pulse energy of 4.3 nJ. Opt. Lett., 33, 690-692(2008).
[14] G. Sobon, J. Sotor, T. Martynkien, K. M. Abramski. Ultra-broadband dissipative soliton and noise-like pulse generation from a normal dispersion mode-locked Tm-doped all-fiber laser. Opt. Express, 24, 6156-6161(2016).
[15] J. H. Lee, J. van Howe, C. Xu, C. Liu. Soliton self-frequency shift: experimental demonstrations and applications. IEEE J. Sel. Top. Quantum Electron., 14, 713-723(2008).
[16] Y. Tang, L. G. Wright, K. Charan, T. Wang, C. Xu, F. W. Wise. Generation of intense 100 fs solitons tunable from 2 to 4.3 μm in fluoride fiber. Optica, 3, 948-951(2016).
[17] G. Krauss, D. Fehrenbacher, D. Brida, C. Riek, A. Sell, R. Huber, A. Leitenstorfer. All-passive phase locking of a compact Er:fiber laser system. Opt. Lett., 36, 540-542(2011).
[18] N. Nishizawa, T. Goto. Widely wavelength-tunable ultrashort pulse generation using polarization maintaining optical fibers. IEEE J. Sel. Top. Quantum Electron., 7, 518-524(2001).
[19] M. Y. Koptev, E. A. Anashkina, A. V. Andrianov, V. V. Dorofeev, A. F. Kosolapov, S. V. Muravyev, A. V. Kim. Widely tunable mid-infrared fiber laser source based on soliton self-frequency shift in microstructured tellurite fiber. Opt. Lett., 40, 4094-4097(2015).
[20] E. A. Anashkina, A. V. Andrianov, M. Y. Koptev, V. M. Mashinsky, S. V. Muravyev, A. V. Kim. Generating tunable optical pulses over the ultrabroad range of 1.6–2.5 μm in GeO2-doped silica fibers with an Er:fiber laser source. Opt. Express, 20, 27102-27107(2012).
[21] J. Sotor, G. Sobon. 24 fs and 3 nJ pulse generation from a simple, all polarization maintaining Er-doped fiber laser. Laser Phys. Lett., 13, 125102(2016).
[22] P. Hlubina, M. Kadulová, D. Ciprian. Spectral interferometry-based chromatic dispersion measurement of fibre including the zero-dispersion wavelength. J. Eur. Opt. Soc., 7, 12017(2012).
[23] J. C. Travers, M. H. Frosz, J. M. Dudley. Supercontinuum Generation in Optical Fibers(2010).
[24] B. Kibler, J. M. Dudley, S. Coen. Supercontinuum generation and nonlinear pulse propagation in photonic crystal fiber: influence of the frequency-dependent effective mode area. Appl. Phys. B, 81, 337-342(2005).
[25] T. Cheng, R. Usaki, Z. Duan, W. Gao, D. Deng, M. Liao, Y. Kanou, M. Matsumoto, T. Misumi, T. Suzuki, Y. Ohishi. Soliton self-frequency shift and third-harmonic generation in a four-hole As2S5 microstructured optical fiber. Opt. Express, 22, 3740-3746(2014).