Abstract
Electrochemical reduction of the greenhouse gas CO2 in solid oxide electrolysis cells (SOECs) has attracted much attention due to their high energy conversion efficiency and great potential for carbon cycling. Compared with the asymmetrical configuration, symmetrical SOECs with the same material as anode and cathode, can greatly simplify the fabrication process and reduce the complication associated with varied interfaces. Perovskite oxides LaxSr2-xFe1.5Ni0.1Mo0.4O6-δ (LxSFNM, x=0.1, 0.2, 0.3 and 0.4) are prepared and evaluated as symmetrical electrodes in solid oxide electrolysis cells for electrochemical reduction of pure CO2. The polarization resistances are 0.07 Ω?cm2 in air and 0.62 Ω?cm2 in 50% CO-50% CO2 for L0.3SFNM electrode at 800 ℃. An electrolysis current density of 1.17 A?cm-2 under 800 ℃ at 1.5 V is achieved for the symmetrical SOECs in pure CO2. Furthermore, the symmetrical cell demonstrates excellent stability during the preliminary 50 h CO2 electrolysis measurements.In the past seventy years, the concentration of CO2 in atmosphere has increased by about 0.012%, which is the main reason of greenhouse effect inducing a series of problems like global warming and climate change[1]. Capture and utilization of CO2 is of great significance to sustainable development of human society. Nevertheless, high thermodynamic stability and kinetic inertness of CO2 limit its conversion and applications[2]. Solid oxide electrolysis cells (SOECs) are one of the most promising technologies to convert CO2 into high energy density fuels and value- added chemicals using the surplus renewable electricity, promoting CO2 utilization and penetration of renewable electricity in the current energy regime as well[3,4].
Ni-based cermets are the commonly used cathodes in SOECs, but suffer from some limitations such as nickel oxidation in CO2-rich atmospheres and carbon deposition in CO-rich atmosphere at high CO2 conversions, which may prevent their practical applications[5,6]. Due to their distinctive features such as brilliant redox stability and excellent resistance against coking formation and sulfur poisoning, mixed ionic-electronic conducting oxides have been widely investigated as alternative cathodes for CO2 electrolysis. Furthermore, some mixed conducting oxides can be simultaneously used as both anodes and cathodes to obtain symmetrical SOECs, which greatly simplifies the fabrication process and reduces complications associated with the different electrode|electrolyte interfaces. Extensive efforts have been made to explore perovskite and double-perovskite electrodes in symmetrical SOECs, such as Sr2Fe1.5Mo0.5O6-δ[7,8], La0.75Sr0.25Cr0.5Mn0.5O3-δ[9], LaxSr1-xTiO3-δ[10,11] and La0.6Sr0.4Fe0.8Ni0.2O3-δ[12]. The symmetrical electrodes should be stable with sufficient electrical conductivities (e.g., >1 S∙cm-1) in both air and fuel environments, and have good chemical compatibility with the other cell components. La0.6Ca0.4Fe0.8Ni0.2O3-δ (LCaFN) was employed as the electrodes for symmetrical SOECs, showing a self-recovery from the performance degradation due to formation of carbonates by air treatment[13]. Sr2Fe1.5Mo0.5O6-δ (SFM), the symmetrical SOFC electrodes developed by Liu, et al. exhibited high electrocatalytic activities for both CO2 reduction and oxygen evolution reactions in symmetrical SOECs[14]. Partial substitution in the A or B site is an effective strategy to optimize the catalytic activity of perovskite oxides. Ce4+ was introduced into the A site of La0.7Sr0.3Cr0.5Fe0.5O3-δ (LSCrF) to increase oxygen vacancies in the lattice by in situ reduction under the operational conditions and thereby enhance the catalytic activities[15]. In this work, La3+ doped Sr2Fe1.5Ni0.1Mo0.4O6-δ (LxSFNM) was investigated as the symmetrical SOEC electrodes for direct CO2 electrolysis. The influence of the La3+ content on the oxygen vacancy concentration, electrical conductivity, and catalytic activity were analyzed. Electrochemical performance was evaluated on symmetrical "LxSFNM@LSGM|LSGM| LxSFNM@LSGM" cells for pure CO2 electrolysis.
1 Experimental
LxSFNM powders were prepared using the Sol-Gel method. The precursor solution was prepared as follows: stoichiometric amounts of the nitrate salts La(NO3)3∙6H2O, Sr(NO3)2, Fe(NO3)3∙9H2O, Ni(NO3)3∙6H2O and (NH4)6Mo7O24∙4H2O were firstly dissolved in distilled water, and then citric acid was added with the molar ratio of the citric acid to the total metal ions at 1.2 : 1. The precursor solution was subsequently heated at 80 ℃ until a gel was formed, followed by drying at 200 ℃ for 5 h and calcination at 1000 ℃ in air for 5 h to obtain pure LxSFNM powders.
The tri-layer structure of “porous|dense|porous” LSGM (La0.9Sr0.1Ga0.8Mg0.2O3-δ) was prepared by laminating three tape-cast green tapes at 75 ℃ and 20 MPa, with 40wt% rice starch and graphite used as the pore-forming material in the porous layers. The laminated layers were co-fired at 1380 ℃ to produce the final ceramic structures. LxSFNM catalysts were added into the porous LSGM scaffolds by impregnating the precursor solution with the total concentration of metal ions at 1 mol∙L-1, followed by calcination at 1000 ℃ for 5 h. The impregnation and calcination cycle was repeated for 16 times so as to achieve an LxSFNM loading of ~25wt% relative to the LSGM scaffolds.
The X-ray diffraction (XRD) pattern of as-synthesized powders was examined at room temperature on an Rigaku D/Max 2100 Powder X-ray Diffractometer with a monochromatic Cu Kα and the diffraction data were recorded in the range 2θ=20o-80o with scan rate of 5 (o)/min. The cell structure was examined using the scanning electron microscope (SEM) in a FEI Magellan 400 microscope. The conductivities of LxSFNM were tested in air and 50% CO-50% CO2 using the DC four-probe method. For fuel cell measurements, silver ink (DAD87, Shanghai Institute of Synthetic Resin) and silver wires was applied on the anode and cathode electrode surface as the current collectors. Impedance measurements were performed on the symmetrical fuel cells in a homogeneous environment of air or 50% CO-50% CO2. The electrolysis performance of the symmetrical fuel cells was evaluated using IM6 Electrochemical Workstation (ZAHNER, Germany), with pure CO2 fed to the cathodes at 50 sccm and ambient air in the anodes.
2 Results and discussion
The XRD patterns of LaxSr2-xFe1.5Ni0.1Mo0.4O6-δ oxides at room temperature are shown in Fig. 1(a). All samples showed a pure cubic perovskite structure without any extra peaks of impurities observed. Fig. 1(b) shows that the (110) peak shifts to a higher scattering angle at x=0.1, which can be explained by smaller ionic radius for La3+ than that for Sr2+ (0.136 nm vs. 0.144 nm). With the La3+ content further increasing, the diffraction peak shifted to lower scattering angles. Prior reports showed that the cell expansion was inhibited due to the steric effects associated with anti-site defects[16,17]. This indicates the existence of a disordered crystal structure in LxSFNM double perovskites.
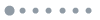
Figure 1.(a) XRD patterns of LxSFNM (x=0-0.4) powder calcined at 1000 ℃ in air for 5 h and (b) corresponding magnified patterns within 2θ=31°-35°
Fig. 2 compares the thermogravimetric curves of LxSFNM powders in air, showing a loss of 1%-1.5% in the powder weight due to release of the lattice oxygen with the temperature increasing from 30 to 900 ℃. Note that the largest weight loss was observed for L0.3SFNM, indicating the most abundant presence of oxygen vacancies at high temperatures. Fig. 3(a, b) show the conductivities of LxSFNM measured in air or 50% CO-50% CO2 at 650-800 ℃. Due to their p-type conducting nature, the conductivities were much higher in air than those in 50% CO-50% CO2 (17.3-38.1 vs. 2.7-5.5 S∙cm-1). Fig. 3 also shows that L0.3SFNM had the highest conductivities among four samples at all temperatures. For x≤0.3, partial substitution of La3+ for Sr2+ increased the concentration of anti-site defects and the electron density of Fermi level. It has been reported that the half metallic properties of SFM was quite sensitive to the anti-site defect concentration as it altered the Fe-O-Mo bonding network and the electronic hopping pathway[18]. Due to a decrease in the crystal symmetry and the cation order, the double exchange interaction between Fe-O-Mo may be inhibited at x≥0.4, leading to decreased conductivities[19,20,21].
Electrode | Electrolyte | Performance/(A∙cm-2)
| Ref. |
---|
La0.3Sr0.7Fe0.7Ti0.3O3 | YSZ | 0.52 (2V) | [27] |
La0.6Sr0.4Fe0.9Mn0.1O3-δ-GDC
| YSZ | 1.107 (2V) | [25] |
La0.6Sr0.4Fe0.8Ni0.2O3-δ-GDC
| YSZ | 1.03 | [28] |
La0.4Sr0.6Co0.2Fe0.7Nb0.1O3-δ | YSZ | 0.442 | [29] |
La0.6Ca0.4Fe0.8Ni0.2O3-δ-GDC
| YSZ | 0.78 | [13] |
La0.75Sr0.25Cr0.5Mn0.5O3-δ | YSZ | 0.09 | [30] |
La0.3Sr0.7Cr0.3Fe0.7O3-δ | YSZ | 0.32 | [31] |
(PrBa)0.95(Fe0.9Mo0.1)2O5+δ | LSGM | 0.51 (1.3V) | [32] |
La0.3Sr1.7Fe1.5Ni0.1Mo0.4O6-δ | LSGM | 1.17 | This work |
Table 1. Comparison of literature values of current densities achieved for pure CO2 electrolysis in electrolyte-supported cells at 1.5 V under 800 ℃
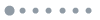
Figure 2.TGA analysis of LxSFNM in air
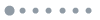
Figure 3.Electricity conductivities of LxSFNM at 650-800 ℃ in (a) air and (b) 50% CO-50% CO2
In order to study the effect of different La3+ contents on the activities of LxSFNM oxides toward oxygen evolution and CO2 reduction reactions, electrochemical impedance measurements were performed on electrolyte- supported symmetrical cells with impregnated LxSFNM electrodes, i.e., LxSFNM@LSGM|LSGM|LxSFNM@LSGM (Fig. 4(a)). The dense LSGM electrolytes were 210 μm in thickness, whereas the porous electrodes were around 20 μm in thickness. Fig. 4(b) shows excellent interfacial bonding between dense electrolytes and the porous scaffolds that is conducive to minimizing the contact resistance. As shown in Fig. 4(c), nano-scale LxSFNM catalysts were deposited onto the internal surfaces of porous LSGM scaffolds to form a well-interconnected network to facilitate electronic conduction and thus enhance their catalytic activities.
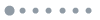
Figure 4.(a) Cross-sectional SEM image of the tri-layer symmetrical structure of “porous|dense|porous” LSGM and (b) high magnification view of symmetrical cell, and (c) high-magnification view of impregnated L0.3SFNM catalyst
Electrochemical impedance measurements were performed for symmetrical cells in the homogeneous environment of dry air or 50% CO-50% CO2, with the typical Nyquist plots of impedance data as compared in Fig. 5. Note that the electrolyte resistances were subtracted from the cell impedance, and the polarization resistances were divided by two due to the symmetrical configurations. Fig. 5(a) shows that the anode polarization resistances (RP,A) at 800 ℃ are 0.12, 0.09, 0.07 and 0.10 Ω∙cm2 for L0.1SFNM, L0.2SFNM, L0.3SFNM and L0.4SFNM in dry air, respectively. L0.3SFNM exhibited much smaller RP,A compared with some previously reported air electrodes, such as Sr2FeMoO6 (0.1 Ω∙cm2 at 850 ℃)[22], Sr2Fe1.4Ni0.1Mo0.5O6-δ (0.22 Ω∙cm2 at 750 ℃)[23], Pr0.6Sr0.4Fe0.7Ni0.1Mo0.1O3-δ (0.4 Ω∙cm2 at 800 ℃)[24] and La0.6Sr0.4Fe0.9Mn0.1O3-δ-GDC (0.24 Ω∙cm2 at 800 ℃)[25]. Distribution of relaxation time (DRT) analyses were conducted on impedance data so as to identify the number of polarization processes involved in the oxygen evolution reaction. With spectra transferred from the frequency into time domain, the overlapped polarization processes can be clearly distinguished. Fig. 5(b) shows that all DRT curves have three distinctive peaks, corresponding to three elementary reaction processes in the oxygen evolution reaction. The low-frequency peaks (1-10 Hz) were usually assigned to gas diffusion in the porous electrodes, while the high frequency peaks (300-2000 Hz) were related to the charge transfer process across the electrode- electrolyte interfaces. RLF and RHF were almost independent of the La3+ content. Note that the intermediate frequency peaks (50-200 Hz) dropped pronouncedly in the order of L0.1SFNM > L0.4SFNM > L0.2SFNM > L0.3SFNM. Given that the intermediate frequency peaks were usually associated with the bulk transport of oxygen surface exchange and O2- bulk diffusion[26], it can be concluded that L0.3SFNM had the highest surface oxygen exchange rate. The highest activity of L0.3SFNM toward oxygen evolution was also consistent with the smallest activation energy of RP,A among the investigated LxSFNM (Fig. 6(a)). It should also be pointed out that the intermediate frequency peaks were much larger than the low- or high-frequency peaks for all LxSFNM samples, indicating that surface oxygen exchange is the rate-limiting step in oxygen evolution reaction.
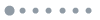
Figure 5.Nyquist plots of impedance data measured with LxSFNM at 800 ℃ in (a) air and (c) 50% CO-50% CO2; (b, d) DRT curves of impedance data shown in (a, c)
Fig. 5(c) shows an order of the cathode polarization resistances (RP,C) - L0.3SFNM (0.62 Ω∙cm2)0.2SFNM (0.86 Ω∙cm2) < L0.4SFNM (0.98 Ω∙cm2) < L0.1SFNM (1.24 Ω∙cm2) measured in 50% CO-50% CO2, demonstrating that L0.3SFNM had the highest activities toward CO2 reduction reactions. Fig. 5(d) shows the DRT analysis results of impedance data in Fig. 5(c), also showing three well distinguished peaks. The low-, intermediate- and high-frequency peaks were associated with dissociative adsorption of CO2 molecules on the surface, surface diffusion and the charge transfer process, respectively. RHF and RIF remained independent of the La3+ content, while RLF showed the lowest at x=0.3. Much higher RLF than RHF and RIF indicated that the overall CO2 electro- reduction was limited by dissociative adsorption of CO2 on the surface of LSFNM. Moreover, the cathode polarization resistances (RP,C) was much larger than RP,A, demonstrating that the electrochemical performance of the CO2 electrolysis cells mainly depended on the catalytic activities of cathodes toward CO2 reduction reactions. Fig. 6(b) shows that the activation energies of RP,C are 0.83-0.89 eV.
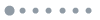
Figure 6.Measured polarization resistances versus the temperature reciprocal in (a) air and (b) 50% CO-50% CO2
The electrochemical performance of L0.3SFNM electrode for pure CO2 electrolysis was further evaluated using the LSGM-electrolyte supported SOECs at 650-800 ℃, with pure CO2 in the cathodes at 50 sccm and ambient air in the anodes. Fig. 7(a) shows the curves of measured voltages as a function of current densities. At the applied voltage of 1.5 V, the electrolysis current densities increased from 0.4 A∙cm-2 at 650 ℃ to 1.17 A∙cm-2 at 800 ℃. These values were competitive when compared with previously reported results listed in Table 1. Fig. 7(b) compares the corresponding EIS spectra measured at 1.5 V. The Ohmic resistance and polarization resistance both increased obviously with reduced cell operating temperature. The interfacial polarization resistances were 0.13 Ω∙cm2 at 800 ℃ and 0.52 Ω∙cm2 at 650 ℃. Fig. 7(c) summarizes the correlation between the doping amount of La3+ ions and the electrolysis current density at 1.5 V, showing that L0.3SFNM yielded the highest performance at all measurement temperatures. Fig. 7(d) shows the preliminary short-term stability of symmetrical L0.3SFNM electrode cells measured at 800 ℃ and 1.3 V in pure CO2. The current density slightly dropped from 0.68 to 0.64 A∙cm-2 during the first 2 h, and then remained stable, indicating good stability of the nano-scale L0.3SFNM electrodes.
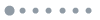
Figure 7.(a) I-V curves and (b) impedance spectra measured at the 1.5 V of a single electrolyte-supported electrolysis cell with L0.3SFNM at different temperatures with inset in (b) showing corresponding enlarged spectrum of the cell with L0.3SFNM at 800 ℃, (c) current densities with varied LaxSFNM anodes at 1.5 V under different temperatures, and (d) short-term stability test of the symmetrical SOECs with L0.3SFNM electrode, operating under 800 ℃ at an applied voltage of 1.3 V
3 Conclusions
In summary, a series of perovskite oxides LaxSr2-xFe1.5Ni0.1Mo0.4O6-δ (LxSFNM, x=0.1, 0.2, 0.3 and 0.4) have been synthesized and evaluated as symmetrical electrodes for solid oxide electrolysis cells. Impedance measurements show that the electrode performance strongly depended upon the La3+ doping content in LxSFNM. Both of the highest activities for oxygen evolution and CO2 reduction observed both at x=0.3. LSGM electrolyte- supported SOECs produce an electrolysis current density of 1.17 A∙cm-2 at 800 ℃ and 1.5 V in pure CO2, and good stability was observed during a preliminary 50 h measurement. These results demonstrate that L0.3SFNM could be a promising alternative as symmetrical electrodes in SOECs for pure CO2 electrolysis.
References
[1] J ALBO, M ALVAREZ-GUERRA, P CASTAÑO et al. Towards the electrochemical conversion of carbon dioxide into methanol. Green Chemistry, 17, 2304-2324(2015). http://xlink.rsc.org/?DOI=C4GC02453B
[2] J FREUND H, W ROBERTS M. Surface chemistry of carbon dioxide. Surface Science Reports, 25, 225-273(1996). https://linkinghub.elsevier.com/retrieve/pii/S0167572996000076
[3] Y ZHENG, J WANG, B YU et al. A review of high temperature co-electrolysis of H2O and CO2 to produce sustainable fuels using solid oxide electrolysis cells (SOECs): advanced materials and technology. Chem. Soc. Rev., 46, 1427-1463(2017). http://xlink.rsc.org/?DOI=C6CS00403B
[4] S LIU, Q LIU, L LUO J. CO2-to-CO conversion on layered perovskite with in situ exsolved Co-Fe alloy nanoparticles: an active and stable cathode for solid oxide electrolysis cells. Journal of Materials Chemistry A, 4, 17521-17528(2016). http://xlink.rsc.org/?DOI=C6TA06365A
[5] V SINGH, H MUROYAMA, T MATSUI et al. Feasibility of alternative electrode materials for high temperature CO2 reduction on solid oxide electrolysis cell. Journal of Power Sources, 293, 642-648(2015). https://linkinghub.elsevier.com/retrieve/pii/S0378775315009908
[6] L YUE X, S IRVINE J T. Alternative cathode material for CO2 reduction by high temperature solid oxide electrolysis cells. Journal of the Electrochemical Society, 159, F442-F448(2012). https://iopscience.iop.org/article/10.1149/2.040208jes
[7] Y LI, X CHEN, Y YANG et al. Mixed-conductor Sr2Fe1.5Mo0.5O6-δ as robust fuel electrode for pure CO2 reduction in solid oxide electrolysis cell. ACS Sustainable Chemistry & Engineering, 5, 11403-11412(2017).
[8] H LÜ, L LIN, X ZHANG et al. In situ investigation of reversible exsolution/dissolution of CoFe alloy nanoparticles in a Co-doped Sr2Fe1.5Mo0.5O6-δ cathode for CO2 electrolysis. Advanced Materials, 32, 1906193(2020). https://onlinelibrary.wiley.com/toc/15214095/32/6
[9] X YUE, S IRVINE J T. Modification of LSCM-GDC cathodes to enhance performance for high temperature CO2 electrolysis using solid oxide electrolysis cells (SOECs). Journal of Materials Chemistry A, 5, 7081-7090(2017). http://xlink.rsc.org/?DOI=C6TA09421J
[10] L LU, C NI, M CASSIDY et al. Demonstration of high performance in a perovskite oxide supported solid oxide fuel cell based on La and Ca co-doped SrTiO3-δ. Journal of Materials Chemistry A, 4, 11708-11718(2016). http://xlink.rsc.org/?DOI=C6TA04074H
[11] W QI, Y GAN, D YIN et al. Remarkable chemical adsorption of manganese-doped titanate for direct carbon dioxide electrolysis. Journal of Materials Chemistry A, 2, 6904-6915(2014). http://xlink.rsc.org/?DOI=C4TA00344F
[12] S LIU, Q LIU, L LUO J. Highly stable and efficient catalyst with in situ exsolved Fe-Ni alloy nanospheres socketed on an oxygen deficient perovskite for direct CO2 electrolysis. ACS Catalysis, 6, 6219-6228(2016). https://pubs.acs.org/doi/10.1021/acscatal.6b01555
[13] Y TIAN, L ZHANG, Y LIU et al. A self-recovering robust electrode for highly efficient CO2 electrolysis in symmetrical solid oxide electrolysis cells. Journal of Materials Chemistry A, 7, 6395-6400(2019). http://xlink.rsc.org/?DOI=C9TA00643E
[14] Y LI, Z ZHAN, C XIA. Highly efficient electrolysis of pure CO2 with symmetrical nanostructured perovskite electrodes. Catalysis Science & Technology, 8, 980-984(2018).
[15] Q ZHANG Y, H LI J, F SUN Y et al. Highly active and redox- stable Ce-doped LaSrCrFeO based cathode catalyst for CO2 SOECs. ACS Applied Materials Interfaces, 8, 6457-6463(2016). https://pubs.acs.org/doi/10.1021/acsami.5b11979
[16] D SÃNCHEZ, A ALONSO J, M GARCÍA-HERNÁNDEZ et al. Microscopic nature of the electron doping effects in the double perovskite Sr2-xLaxFeMoO6(0≤x≤1) series. Journal of Materials Chemistry A, 13, 1771-1777(2003).
[17] T SUGAHARA, M OHTAKI, T SOUMA. Thermoelectric properties of double-perovskite oxide Sr2-xMxFeMoO6 (M=Ba, La). Journal of Ceramic Society Japan, 116, 1278-1282(2008). https://www.jstage.jst.go.jp/article/jcersj2/116/1360/116_1360_1278/_article
[18] X YANG, J CHEN, D PANTHI et al. Electron doping of Sr2FeMoO6-δ as high performance anode materials for solid oxide fuel cells. Journal of Materials Chemistry A, 7, 733-743(2019). http://xlink.rsc.org/?DOI=C8TA10061F
[19] F AZIZI, A KAHOUL, A AZIZI. Effect of La doping on the electrochemical activity of double perovskite oxide Sr2FeMoO6 in alkaline medium. Journal of Alloys and Compounds, 484, 555-560(2009). https://linkinghub.elsevier.com/retrieve/pii/S0925838809009074
[20] X YANG, D PANTHI, N HEDAYAT et al. Molybdenum dioxide as an alternative catalyst for direct utilization of methane in tubular solid oxide fuel cells. Electrochemistry Communications, 86, 126-129(2018). https://linkinghub.elsevier.com/retrieve/pii/S1388248117303399
[21] D SARMA D, P MAHADEVAN, S DASGUPTA T et al. Electronic structure of Sr2FeMoO6-δ. Physical Review Letter, 85, 2549-2552(2000). https://link.aps.org/doi/10.1103/PhysRevLett.85.2549
[22] Q LIU, X DONG, G XIAO et al. A novel electrode material for symmetrical SOFCs. Advanced Materials, 22, 5478-5482(2010). http://doi.wiley.com/10.1002/adma.v22.48
[23] N DAI, J FENG, Z WANG et al. Synthesis and characterization of B-site Ni-doped perovskites Sr2Fe1.5-xNixMo0.5O6-δ (x = 0, 0.05, 0.1, 0.2, 0.4) as cathodes for SOFCs. Journal of Materials Chemistry A, 1, 14147-14153(2013). http://xlink.rsc.org/?DOI=c3ta13607h
[24] X LU, Y YANG, Y DING et al. Mo-doped Pr0.6Sr0.4Fe0.8Ni0.2O3-δ as potential electrodes for intermediate-temperature symmetrical solid oxide fuel cells. Electrochimica Acta, 227, 33-40(2017). https://linkinghub.elsevier.com/retrieve/pii/S0013468616327293
[25] X PENG, Y TIAN, Y LIU et al. An efficient symmetrical solid oxide electrolysis cell with LSFM-based electrodes for direct electrolysis of pure CO2. Journal of CO2 Utilization, 36, 18-24(2020).
[26] R WANG, E DOGDIBEGOVIC, Y LAU G et al. Metal-supported solid oxide electrolysis cell (MS-SOEC) with significantly enhanced catalysis. Energy Technology, 7, 1801154-1801166(2019). https://onlinelibrary.wiley.com/toc/21944296/7/5
[27] Z CAO, B WEI, J MIAO et al. Efficient electrolysis of CO2 in symmetrical solid oxide electrolysis cell with highly active La0.3Sr0.7Fe0.7Ti0.3O3 electrode material. Electrochemistry Communications, 69, 80-83(2016). https://linkinghub.elsevier.com/retrieve/pii/S1388248116301369
[28] Y TIAN, H ZHENG, L ZHANG et al. Direct electrolysis of CO2 in symmetrical solid oxide electrolysis cell based on La0.6Sr0.4Fe0.8Ni0.2O3-δelectrode. Journal of The Electrochemical Society, 165, F17-F23(2018). https://iopscience.iop.org/article/10.1149/2.0351802jes
[29] Z YANG, C MA, N WANG et al. Electrochemical reduction of CO2 in a symmetrical solid oxide electrolysis cell with La0.4Sr0.6Co0.2Fe0.7Nb0.1O3-δ electrode. Journal of CO2 Utilization, 33, 445-451(2019).
[30] S XU, S LI, W YAO et al. Direct electrolysis of CO2 using an oxygen-ion conducting solid oxide electrolyzer based on La0.75Sr0.25Cr0.5Mn0.5O3-δ electrode. Journal of Power Sources, 230, 115-121(2013). https://linkinghub.elsevier.com/retrieve/pii/S0378775312019143
[31] K ADDO P, B MOLERO-SANCHEZ, M CHEN et al. CO/CO2 study of high performance La0.3Sr0.7Fe0.7Cr0.3O3-δ reversible SOFC electrodes. Fuel Cells, 15, 689-696(2015). http://doi.wiley.com/10.1002/fuce.v15.5
[32] C LU, B NIU, W YI et al. Efficient symmetrical electrodes of PrBaFe2-xCoxO5+δ (x=0, 0.2, 0.4) for solid oxide fuel cells and solid oxide electrolysis cells. Electrochimica Acta, 358, 136916-136927(2020). https://linkinghub.elsevier.com/retrieve/pii/S0013468620313098