
- Matter and Radiation at Extremes
- Vol. 6, Issue 6, 068201 (2021)
Abstract
I. INTRODUCTION
Room-temperature superconductivity has been one of the most attractive targets in condensed matter physics ever since Kamerlingh Onnes found the superconducting transition of Hg at 4.2 K in 1911.1 However, the highest superconducting transition temperature TC that can be achieved by cuprates, the most representative class of unconventional superconductors, is 135 K at ambient pressure,2 and even at high pressure only reaches as high as 160 K.3 On the other hand, it has been proposed that under high pressure, solid hydrogen should achieve metallization and high-TC superconductivity (100–760 K) in either molecular4–6 or atomic phases,7,8 based on Bardeen–Cooper–Schrieffer (BCS) theory,9 although the pressure required is far beyond what can be experimentally achieved.10,11 Excitingly, however, hydrides are predicted to be able to achieve high-TC superconductivity at relatively low pressure owing to chemical precompressions,12 and this has led to an upsurge in research on compressed hydrides.
Thus far, remarkable achievements have been made with pressure-induced superconductivity in hydrides,13 bringing room-temperature superconductivity within reach. In terms of element category, almost all of the known binary hydrides have been studied theoretically or experimentally.14–20 On the other hand, a plethora of ternary hydrides exhibiting superconductivity are also being explored, and there is a broad development space in terms of diverse chemical compositions, synergistic charge transfer, and combinations of the merits of different elements.21–38
As proposed by Ashcroft,12 hydrides can achieve superconductivity under much lower pressures than metallic hydrogen. Following this principle, numerous hydrides are predicted to have TC values above 200 K,18,39–42 with some of them exhibiting superconductivity near room temperature and even higher.21,22,43 Even more interestingly, these high-TC hydrides (>200 K) contain diverse hydrogen configurations, such as isolated atomic hydrogen in covalent hydrides, two-dimensional (2D) H motifs, and various 3D H cages with covalent H–H bonding character.
It is worth noting that theoretical calculations play a crucial role in identifying conventional superconductors such as H3S,44,45 LaH10,46–49 YH9,46,50 and PrH9,51 and provide effective guidance for experimental synthesis.52,53 Meanwhile, more and more advanced prediction methods are being developed, inevitably accelerating the development of hydride superconductors.54–61 Theoretical calculations also have unique advantages in revealing the microscopic mechanism of superconductivity from both physical and chemical aspects, including chemical bonding, charge distribution, and electronic properties, as well as quantum effects.62–65
To gain insight into the features of high-TC hydrides and provide inspiration for research on superconductors, in this review, the reported hydrides with TC above 200 K are summarized with regard to their crystal structures, chemical bonds, electronic properties, and origin of superconductivity. The focus is mainly on covalent H3S and several clathrate superhydrides (LaH10, YH9, and CaH6) that have been predicted by theory and verified by experiment, although other theoretically predicted hydrides with clathrate H cages and 2D H motifs are also described. Finally, a comprehensive discussion and conclusions are presented, including a description of current problems and future challenges.
II. HIGH-PRESSURE COVALENT HYDRIDES
High-pressure covalent hydrides with TC above 200 K are exemplified by Im-3m H3S. The good agreement between experimental measurements45,66 and theoretical calculations44 provides a firm basis for the exploration of pressure-induced superconducting hydrides and for unveiling the mechanism of their superconductivity. In another important development, it has been demonstrated that the TC value of H3S can be significantly elevated via hole-doping.43,67
The high-TC superconductivity of H3S was observed in a high-pressure experiment aimed at verifying the predicted superconductivity of H2S.45 The TC of 203 K and its pressure dependence are in good agreement with the theoretical prediction for Im-3m H3S,44 thus attracting great research interest. Im-3m H3S contains body-centered cubic (bcc) S sites, and a nested [H3S] sublattice in which each H atom bonds symmetrically with two S atoms forming a S-centered SH6 octahedron [Fig. 1(a)], indicating the presence of atomic hydrogen.68 Based on the weights of atomic orbitals, the large overlap of H s and S 3p orbitals below the Fermi level EF indicates significant hybridization between them and the formation of a strong polar S–H covalent bond [Fig. 1(b)], which is consistent with the calculated electron localization function.44 From its calculated electronic properties, H3S is a good metal with a large density of states (DOS) peak near EF and a band characterization of “flat band–steep band” [Fig. 1(b)]. Further, the Fermi surface for the single band contributing the main DOS near the EF shows significant pockets [colored by the Fermi velocity in Fig. 1(c)].64 These results demonstrate that Im-3m H3S is a potential covalent metallicity-driven conventional superconductor. As expected, Im-3m H3S is calculated to have strong electron–phonon coupling (EPC) with λ = 2.19, a large logarithmic average phonon frequency ωlog of 1334.6 K, and a high TC value of 204 K at 200 GPa (μ* = 0.1). From the corresponding phonon density of states (PHDOS) and Eliashberg spectral function a2F(ω) [Fig. 1(d)], such a high TC mainly originates from the large EPC contribution (82.6%) of a high-frequency hydrogen vibrational mode (>20 THz).44
Figure 1.(a) Crystal structure of
Great effort has been made to further verify whether Im-3m H3S is the source of high-TC superconductivity in compressed H2S. Extensive structural searches for S–H system with different stoichiometries indicate that H2S becomes unstable and decomposes into H3S and elemental β-Po sulfur above 43 GPa.64,69 Specifically, pressure-induced metallic H3S shows a phase transition from trigonal R3m to cubic Im-3m at 180 GPa. Moreover, the two phases of H3S are estimated to have TC values of 155–166 K for the R3m phase at 130 GPa and 191–204 K for the Im-3m structure at 200 GPa.44 These theoretical results have been confirmed by synchrotron x-ray diffraction (XRD) combined with electrical resistance measurements [Figs. 1(e)–1(g)].66 Therefore, the conclusion has been reached that cubic Im-3m H3S is the primary contributor to the high TC above 200 K in compressed H2S owing to the metallicity driven by strong covalent bonds and the stabilization of atomic hydrogen by sulfur.64,68 In addition, the quantum nature of the proton has a crucial effect on the stable pressure and symmetric hydrogen bonds in Im-3m H3S.63
As an effective method to modulate superconductivity of materials,70–73 atomic substitution is also performed to elevate the TC of H3S. The results demonstrate that partial substitution of S with P, C, and Si atoms significantly enhances the TC to above 280 K.43,67 In detail, the PDOS of Im-3m H3S exhibits a conspicuous van Hove singularity below EF [Fig. 1(b)], implying that hole doping probably moves the van Hove singularity to EF, thus increasing the DOS at EF and strengthening EPC. This can be realized by partial substitution of three kinds of atoms (e.g., P,67 C,43 and Si43) with fewer valence electrons than an S atom. Moreover, the DOS at EF and the EPC constant first increase and then decrease with increasing doping content [Figs. 1(h), 1(i), and 1(k)]. Near room-temperature superconductivity is realized in H3S0.925P0.075 (TC = 280 K at 250 GPa),67 H3S0.962C0.038 (TC = 289 K at 260 GPa), and H3S0.960Si0.040 (TC = 283 K at 230 GPa) [Figs. 1(j) and 1(l)].67 Note that the pressure-dependent TC values for P/C/Si-doped H3S indicate that application of an appropriate pressure is also an important way to maximize the TC of doped H3S [Figs. 1(j) and 1(l)]. On the other hand, the high-TC superconductivity of H3S0.962C0.038 also provides a possible explanation for the recent experimental observation of room-temperature superconductivity in a highly compressed C–S–H system [Fig. 1(m)].22
III. CLATHRATE SUPERHYDRIDES
A. Experimentally verified LaH10, YH9, and CaH6
Clathrate superhydrides comprise another important class of superconductors with TC above 200 K. Typical representatives are Fm-3m LaH10,47,48P63/mmc YH9,50 and Im-3m CaH6,74 which have been synthesized with direct guidance from theoretical predictions.46,49,75 Their synthesis not only opens a potential route to achieving room-temperature superconductivity in clathrate superhydrides, but also indicates that other predicted high-TC clathrate superhydrides might be successfully synthesized.
Fm-3m LaH10 is predicted to adopt a sodalite-like face-centered cubic (fcc) structure, in which each La atom is surrounded by a cage of 32 H atoms [Fig. 2(a)].49 Each H-cage consists of 6 H-square and 12 H-hexagon rings, with two inequivalent H atoms, named H1 and H2. The H–H bond length is close to that of the predicted atomic metallic hydrogen near 500 GPa (1.0 Å),9 indicating that H atoms are bonded to each other with covalent bonds.65 As a result, all H vibrations can effectively participate in the EPC process, leading to strong EPC (λ = 2.2) and high TC values of 257–274 K with μ* = 0.1–0.13 at 250 GPa.49 This prediction has been verified by two experimental groups,46,47 who have reported an La hydride LaH10±x (x < 1) with TC ≈ 260 K at about 190 GPa or 250 K at about 170 GPa [Fig. 2(e)].
Figure 2.(a) Structure of LaH10.
Furthermore, the bonding nature and superconducting mechanism of LaH10 have been extensively investigated. Intuitively, La atoms are thought to transfer charge to H atoms, suppressing the formation of H2 molecules (extra charges occupy the antibonding orbitals).49 However, based on the charge density of LaH10, besides the H–H bonds, the La–H1 bonds also exhibit covalent character from the connected charges between La and H1 atoms [Fig. 2(b)]. The charge density difference also indicates charge accumulation in these regions.65 In addition, on removing all H atoms in LaH10, it was found that the metal framework of La atoms generates excess electrons in the interstitial regions, and transfers interstitial electrons to H cages, facilitating stabilization of LaH10.76,77 Therefore, the bonding nature between La atoms and H32 cages is characterized as mixed ionic–covalent bonding.76
On the other hand, there are two van Hove singularities with a separation of ∼90 meV near EF [Fig. 2(c)], originating from the hole-like and electron-like bands [Fig. 2(d)]. Specifically, the two bands arise from the splitting of the symmetry-protected topological states at the equivalent high-symmetry L points.65 A high H-derived DOS at EF and strong hybridization between La f and H1s orbitals are manifested in the DOS and band structure [Figs. 2(c) and 2(d)]. Moreover, the four Fermi surfaces corresponding to four bands crossing EF exhibit strong coupling between the hybridized states (La and H1 atoms as well as H1 and H2 atoms) and the phonon modes in the whole frequency range, giving rise to two nodeless, anisotropic superconducting gaps.78 Consequently, the unusual bonding and electronic properties induce a highly optimized electron–phonon interaction that favors coupling to high-frequency hydrogen phonons,79 and H-dominated high-TC superconductivity in Fm-3m LaH10.80 In addition, the pressure dependence of EPC mainly accounts for the decrease in TC with pressure,80,81 supporting the experimental measurements.47 Notably, these characteristics are very similar to those of Im-3m H3S, indicating some common features of high-TC hydrides. Besides, as in H3S, the inclusion of quantum effects in LaH10 brings the theoretically stable pressure and TC values into better consistency with experimental measurements [Fig. 2(f)].62
Similar to Fm-3m LaH10, several other pressure-induced superhydrides with H32 cages and the same symmetry have also been predicted to exhibit strong H-dominated EPC and high-TC superconductivity above 200 K, such as YH10 with TC = 303–326 K at 250–400 GPa,46,49 Sc- or Y-substituted LaH10 with TC enhanced compared with LaH10,82 and TbH10 with TC > 270 K at 250 GPa,83 although these await experimental confirmation. However, not all LaH10-type superhydrides have TC above 200 K (e.g., TC < 56 K for CeH1046,84 and TC < 82 K for UH1085,86), indicating that the particular metal atom has a vital effect on TC even in the same hydrogen lattice framework.
Encouragingly, another predicted high-TC clathrate superhydride, P63/mmc YH9, has recently been synthesized.46,50 In contrast to LaH10, in P63/mmc YH9, the Y atom is located in an H29 cage, consisting of six H-square, H-pentagon, and H-hexagon rings [Fig. 2(g)]. This densely packed arrangement leads to a much-reduced ΔPV term, stabilizing the structure. The large DOS contributed by Y d and H s orbitals and two van Hove singularities near EF induce strong EPC (λ = 4.42) and a high TC value of 276 K (μ* = 0.1) at 150 GPa [Fig. 2(h)], which has been unambiguously verified in a recent experiment50 [Fig. 2(i)]. Note that the Y atoms contribute a large EPC fraction of 45%. Interestingly, P63/mmc ScH9 is predicted to have a lower TC (<200 K at 120 GPa) than YH9, although Sc has a lower atomic weight than Y. On the other hand, PrH951 and ThH9,87 which are isostructural to YH9, also have much lower TC values of 8.9 K at 120 GPa and 145 K at 150 GPa, respectively.
Beside LaH10-type and YH9-type structures, there is also a class of experimentally verified high-TC clathrate superhydrides with a sodalite-like bcc structure with Im-3m symmetry. In fact, CaH6 with Im-3m symmetry is the first predicted clathrate superhydride with TC > 200 K.75 In this kind of structure, the metal atoms possess a bcc configuration, and are accommodated in H24 cages consisting of six H-square and eight H-hexagon rings [Fig. 3(a)]. A high phonon frequency and large DOS at EF induce strong H-dominated EPC [Fig. 3(b)] and high TC, with examples including CaH6 with TC of 220–235 K at 150 GPa,75 MgH6 with TC of about 260 K above 300 GPa,88 and YH6 with TC of 251–264 K at 120 GPa.89 Again, Im-3m ScH6 has a lower TC (169 K at 350 GPa) than YH6.90 What is noteworthy is that CaH6 and YH6 have been successfully synthesized,74,91 as demonstrated by the XRD and temperature-dependent electrical resistances [Figs. 3(c)–3(g)]. These remarkable achievements provide an effective route to develop more high-TC superconductors through combining theoretical predictions with experimental syntheses. More interestingly, two phases (namely, Pm-3m and Fd-3m) of CaYH12, with the same hydrogen cage as CaH6, are predicted to have TC values up to 230 and 258 K, respectively.92,93
Figure 3.(a) Structure of CaH6.
B. Other theoretically predicted clathrate superhydrides
In addition to the three classes of clathrate superhydrides described above (i.e., LaH10-type with H32 cage,49 YH9-type with H29 cage,46 and CaH6-type with H24 cage75), other varieties of clathrate superhydrides are also predicted to exhibit high-TC superconductivity above 200 K. One outstanding example is the recently predicted Fd-3m Li2MgH16.21 Based on the idea that electron doping is expected to lead to occupation of the antibonding orbital of H–H covalent bonds and the breakup of H2 molecules, Li is introduced into binary MgH16 with large numbers of H2 units, achieving atomic hydrogen cages [Fig. 3(g)]. Each Li atom is surrounded by one H18 cage, which is opened to connect to neighboring H18 cages. Each Mg atom is situated in one closed H28 cage. It has been proposed that a pyrochlore-type Li framework provides electrons to H-cages by forming electrides, not only stabilizing the clathrate H cages but also enhancing the H-dominated DOS at EF.77 As a result, Li2MgH16 is predicted have strong EPC (λ = 3.35) and a superhigh TC of 351 K at 300 GPa [Fig. 3(h)] and even as high as ∼473 K at 250 GPa.
Despite being in the same group as La, the reaction of Ac with H2 is predicted to result in two stable high-TC compositions, namely, AcH10 and AcH16.94R-3m AcH10 with H32 cages is in sharp contrast with Fm-3m LaH10 [Fig. 3(i)]. The shortest H–H distance in R-3m AcH10 is 1.07 Å at 150 GPa. For P-6m2 AcH16 with higher H content, each Ac atom is surrounded by 12 H atoms and 6 H2 molecules with an H–H distance of 0.87 Å at 150 GPa [Fig. 3(j)]. Meanwhile, the H atoms form hexagonal H12 rings in the ab plane. In fact, in the bond length range of <1.3 Å, all H atoms form two kinds of cages: one is the empty H14 cage, and the other is the opened H36 cage surrounding the Ac atom. The two structures are estimated to exhibit strong EPC and high TC values of 251 K at 200 GPa for AcH10 and 241 K at 150 GPa for AcH16 [Fig. 3(k)]. In addition, their TC values decrease monotonically with pressure.94
IV. TWO-DIMENSIONAL HYDROGEN CONFIGURATION
Just as a cage structure is not the only structural motif that can support strong EPC, a 2D hydrogen configuration has also been predicted to lead to high-TC superconductivity, such as in the Cmca metallic phase of solid hydrogen with TC = 242 K at 450 GPa.4 To date, two types of superhydrides with 2D hydrogen configuration have also been predicted to have high TC values above 200 K, examples of which include SrH1095 and HfH10.96
Similar to solid Cmca hydrogen [Fig. 4(a)], SrH10 also has puckered honeycomb H layers with H–H bond lengths between 0.998 and 1.011 Å, with Sr atoms being inserted into the interlayers [Fig. 4(b)].95 The continuous phonon modes and Eliashberg spectral function in the whole frequency range [Fig. 4(c)] induce strong EPC (λ = 3.08) and a high TC of 259 K at 300 GPa. On the other hand, it has recently been proposed that a planar pentagraphene-like hydrogen motif can be stabilized by Hf, Zr, Sc, or Lu atoms, forming novel 2D H10 units via H–H covalent bonds [Fig. 4(d)].96 The metal atoms act as a precompressor and electron donor to the hydrogen sublattice. Metal d and H s and p orbitals are the main contributors to the DOS and generate distinct van Hove singularities, resulting in a large total DOS at EF comparable to that of LaH10 [Fig. 4(e)]. Therefore, high-TC superconductivity induced by strong EPC is obtained theoretically in HfH10 (TC = 234 K at 250 GPa) and ZrH10 (TC = 220 K at 250 GPa). Comparison with YH10 and LaH10 indicates that the high TC values in these hydrides mainly originate from the interaction of electrons with optical phonons and the high DOS at EF associated with H atoms [Fig. 4(f)]. By contrast, ScH10 and LuH10 are predicted to have lower TC values of 158 K at 250 GPa and 152 K at 200 GPa, respectively.96
Figure 4.(a)
V. DISCUSSION AND CONCLUSION
On the whole, as predicted by Ashcroft,12 a large number of hydrides have been found to exhibit high-TC superconductivity above 200 K at lower pressures than are required for metallic hydrogen. Specifically, more and more theoretical predictions have been verified experimentally. In terms of the modes of arrangement of hydrogen atoms, these high-TC hydrides (>200 K) contain different hydrogen configurations such as isolated hydrogen atoms in covalent hydrides, 2D planar and puckered hydrogen motifs, and 3D diverse hydrogen cages. In spite of the appearance of a variety of hydrogen configurations, one common feature of these hydrides is that all the hydrogen atoms form covalent bonds with hydrogen or other atoms, inducing significant H-contributed metallicity, strong EPC, and high-TC superconductivity.
On the other hand, hydrogen is the simplest atom, and exhibits explicit bonding behavior at ambient pressure, but its bonding mechanism under high pressure is rather complex and even unconventional. For instance, how does pressure induce H atoms to form various cages via covalent bonding? These H configurations are completely different from those in the molecular phase at ambient pressure. How do different metal atoms affect H–H covalent bonds and superconductivity in the same hydrogen lattice framework? How do different metal atoms give rise to the formation of various hydrogen sublattices? These questions pose new challenges to high-pressure physics and chemistry.
The discovery of various binary hydrides with TC above 200 K has provided a great stimulus to research into how these critical temperatures can be further raised via doping/substitution, as well as into ternary hydride superconductors. Exploration of ternary hydrides will surely lead to the prediction and synthesis of yet more high-TC superconductors. The advances achieved with high-TC hydrides not only indicate the great potential for achieving room-temperature superconductivity, but also provide a vast arena in which to study the formation mechanisms and physicochemical properties of novel hydrides under extreme conditions.
With the development of advanced synthetic methods and measurement techniques, as well as new theoretical methods, the day on which room-temperature superconductivity is finally achieved is getting closer. From the perspective of practical applications, the urgent task is to reduce the pressure required for stability of the hydrides while maintaining their high TC values. On the other hand, the search for new structural types of superconductors provides an impetus for the development and enrichment of condensed matter theory.
ACKNOWLEDGMENTS
Acknowledgment. The authors acknowledge funding support from the Natural Science Foundation of China under Grant Nos. 21873017 and 21573037, the Postdoctoral Science Foundation of China under Grant No. 2013M541283, the Natural Science Foundation of Hebei Province (Grant No. B2021203030), and the Natural Science Foundation of Jilin Province (Grant No. 20190201231JC).
References
[1] H.Kamerlingh Onnes. The superconductivity of mercury. Comm. Phys. Lab. Univ. Leiden, 122, 122(1911).
[2] L.Beauvais, F.Chen, C. W.Chu, L.Gao, Z. J.Huang, J. G.Lin, R. L.Meng, Y. Y.Sun, Y. Y.Xue. Study of superconductivity in the Hg-Ba-Ca-Cu-O system. Physica C, 213, 261(1993).
[3] F.Chen, C. W.Chu, J. H.Eggert, L.Gao, H. K.Mao, R. L.Meng, D.Ramirez, Q.Xiong, Y. Y.Xue. Superconductivity up to 164 K in HgBa2Ca
[4] A.Continenza, P.Cudazzo, A.Floris, E. K. U.Gross, S.Massidda, G.Profeta, A.Sanna. Ab initio description of high-temperature superconductivity in dense molecular hydrogen. Phys. Rev. Lett., 100, 257001(2008).
[5] T.Cui, Z.He, Q.Li, Y.Ma, Y.Niu, Y.Wang, L.Zhang, G.Zou. Ab initio prediction of superconductivity in molecular metallic hydrogen under high pressure. Solid State Commun., 141, 610(2007).
[6] H. Y.Geng. Public debate on metallic hydrogen to boost high pressure research. Matter Radiat. Extremes, 2, 275(2017).
[7] D. M.Ceperley, J. M.McMahon. High-temperature superconductivity in atomic metallic hydrogen. Phys. Rev. B, 84, 144515(2011).
[8] P.Dalladay-Simpson, E.Gregoryanz, R. T.Howie, C.Ji, B.Li, H.-K.Mao. Everything you always wanted to know about metallic hydrogen but were afraid to ask. Matter Radiat. Extremes, 5, 038101(2020).
[9] N. W.Ashcroft. Metallic hydrogen: A high-temperature superconductor?. Phys. Rev. Lett., 21, 1748(1968).
[10] A.Continenza, P.Cudazzo, A.Floris, E. K. U.Gross, S.Massidda, G.Profeta, A.Sanna. Electron-phonon interaction and superconductivity in metallic molecular hydrogen. II. Superconductivity under pressure. Phys. Rev. B, 81, 134506(2010).
[11] D. M.Ceperley, J. M.McMahon. Erratum: High-temperature superconductivity in atomic metallic hydrogen. Phys. Rev. B, 85, 219902(2012).
[12] N. W.Ashcroft. Hydrogen dominant metallic alloys: High temperature superconductors?. Phys. Rev. Lett., 92, 187002(2004).
[13] L.Han-Yu, M.Yan-Ming, S.Ying. Progress on hydrogen-rich superconductors under high pressure. Acta Phys. Sin., 70, 017407(2021).
[14] R.Arita, L.Boeri, M.Eremets, J. A.Flores-Livas, G.Profeta, A.Sanna. A perspective on conventional high-temperature superconductors at high pressure: Methods and materials. Phys. Rep., 856, 1(2020).
[15] I. A.Kruglov, A. G.Kvashnin, A. R.Oganov, I. A.Savkin, D. V.Semenok. On distribution of superconductivity in metal hydrides. Curr. Opin. Solid State Mater. Sci., 24, 100808(2020).
[16] G.Gao, X.Li, Y.Li, Y.Ma, H.Wang. Hydrogen-rich superconductors at high pressures. Wiley Interdiscip. Rev.: Comput. Mol. Sci., 8, e1330(2018).
[17] M.Chen, Y.Feng, W. J.Li, H. Y.Lv, C. L.Yang, G. H.Zhong. Superconductivity of light-metal hydrides. J. Chin. Chem. Soc., 66, 1246(2019).
[18] U.Pinsook. In search for near-room-temperature superconducting critical temperature of metal superhydrides under high pressure: A review. J. Met., Mater. Miner., 30, 31(2020).
[19] J.Chen, W.Cui, K.Gao, J.Hao, J.Kuang, Y.Li, J.Ma, J.Shi. Metal-element-incorporation induced superconducting hydrogen clathrate structure at high pressure. Chin. Phys. Lett., 38, 027401(2021).
[20] T.Cui, D.Duan, B.Liu, Y.Liu, Y.Ma, Z.Shao. Structure and superconductivity of hydrides at high pressures. Natl. Sci. Rev., 4, 121(2016).
[21] H.Liu, J.Lv, Y.Ma, Y.Sun, Y.Xie. Route to a superconducting phase above room temperature in electron-doped hydride compounds under high pressure. Phys. Rev. Lett., 123, 097001(2019).
[22] N.Dasenbrock-Gammon, M.Debessai, R. P.Dias, K. V.Lawler, R.McBride, A.Salamat, E.Snider, K.Vencatasamy, H.Vindana. Room-temperature superconductivity in a carbonaceous sulfur hydride. Nature, 586, 373(2020).
[23] D.Chandra, R. J.Hemley, T.Muramatsu, M.Somayazulu, T. A.Strobel, V. V.Struzhkin, E.Vinitsky, W. K.Wanene. Metallization and superconductivity in the hydrogen-rich ionic salt BaReH9. J. Phys. Chem. C, 119, 18007(2015).
[24] H.Liu, G.Yang, S.Zhang, L.Zhu. Structure and electronic properties of Fe2SH3 compound under high pressure. Inorg. Chem., 55, 11434(2016).
[25] L.Boeri, C.Kokail, W.von der Linden. Prediction of high-
[26] T.Cui, D.Duan, X.Huang, D.Li, B.Liu, H.Liu, Y.Ma, Z.Shao, F.Tian, H.Yu. Divergent synthesis routes and superconductivity of ternary hydride MgSiH6 at high pressure. Phys. Rev. B, 96, 144518(2017).
[27] N. W.Ashcroft, R.Hoffmann, M.Rahm. Ternary gold hydrides: Routes to stable and potentially superconducting compounds. J. Am. Chem. Soc., 139, 8740(2017).
[28] T.Cui, D.-F.Duan, D.Li, B.-B.Liu, Y.Liu, Z.Liu, F.-B.Tian, S.-L.Wei. Pressure-induced superconducting ternary hydride H3SXe: A theoretical investigation. Front. Phys., 13, 137107(2018).
[29] A.Bergara, X.Du, J.Lin, G.Yang, S.Zhang, X.Zhang. Phase diagrams and electronic properties of B-S and H-B-S systems under high pressure. Phys. Rev. B, 100, 134110(2019).
[30] N. N.Degtyarenko, K. S.Grishakov, E. A.Mazur. Electron, phonon, and superconducting properties of yttrium and sulfur hydrides under high pressures. J. Exp. Theor. Phys., 128, 105(2019).
[31] A.Bergara, G.Gao, Y.Gao, J.He, X.Liang, H.Liu, C.Shao, R.Sun, Y.Tian, L.Wang, D.Yu, Y.Zhang, S.Zhao, Z.Zhao, X.-F.Zhou. First-principles study of crystal structures and superconductivity of ternary YSH6 and LaSH6 at high pressures. Phys. Rev. B, 100, 184502(2019).
[32] Y.Iijima, D.Meng, S.-i.Orimo, H.Saitoh, M.Sakata, T.Sato, K.Shimizu, S.Takagi. Superconductivity of the hydrogen-rich metal hydride Li5MoH11 under high pressure. Phys. Rev. B, 99, 024508(2019).
[33] L.Boeri, S.Di Cataldo, W.von der Linden. Phase diagram and superconductivity of calcium borohyrides at extreme pressures. Phys. Rev. B, 102, 014516(2020).
[34] H.-L.Chen, X.Guo, K. M.Ho, W.-C.Lu, C. Z.Wang, R.-L.Wang. Stability and superconductivity of TiPHn (n = 1–8) under high pressure. Phys. Lett. A, 384, 126189(2020).
[35] C.Chen, P.Huang, X.Li, H.Liu, Y.Ma, Y.Sun, Y.Xie. Chemically tuning stability and superconductivity of P–H compounds. J. Phys. Chem. Lett., 11, 935(2020).
[36] T.Iitaka, B.Jiang, H.Li, X.Li, Y.Sun, Y.Tian, Y.Xie, X.Zhong. Computational discovery of a dynamically stable cubic SH3-like high-temperature superconductor at 100 GPa via CH4 intercalation. Phys. Rev. B, 101, 174102(2020).
[37] T.Bi, N.Geng, X.Wang, Y.Yan, E.Zurek. A metastable CaSH3 phase composed of HS honeycomb sheets that is superconducting under pressure. J. Phys. Chem. Lett., 11, 9629(2020).
[38] J.Bang, H.Hosono, J.-Y.Hwang, S. W.Kim, S.-G.Kim, Y.Kim, K.Lee, K. H.Lee, S. Y.Lee, Y. H.Lee, Y.Ma, C. N.Nandadasa, J.Park, Y.Zhang. Ferromagnetic quasi-atomic electrons in two-dimensional electride. Nat. Commun., 11, 1526(2020).
[39] A. P.Drozdov, M. I.Eremets. High-temperature conventional superconductivity. Phys.-Usp., 59, 1154(2016).
[40] J. S.Tse, Y.Yao. Superconducting hydrogen sulfide. Chem. - Eur. J., 24, 1769(2018).
[41] T.Bi, E.Zurek. High-temperature superconductivity in alkaline and rare earth polyhydrides at high pressure: A theoretical perspective. J. Chem. Phys., 150, 050901(2019).
[42] G. B.Bachelet, L.Boeri. Viewpoint: The road to room-temperature conventional superconductivity. J. Phys.: Condens. Matter, 31, 234002(2019).
[43] R. P.Dias, Y.Ge, R. J.Hemley, Y.Yao, F.Zhang. Hole-doped room-temperature superconductivity in H3S1−
[44] T.Cui, D.Duan, X.Huang, D.Li, B.Liu, Y.Liu, F.Tian, W.Tian, H.Yu, Z.Zhao. Pressure-induced metallization of dense (H2S)2H2 with high-
[45] A. P.Drozdov, M. I.Eremets, V.Ksenofontov, S. I.Shylin, I. A.Troyan. Conventional superconductivity at 203 kelvin at high pressures in the sulfur hydride system. Nature, 525, 73(2015).
[46] Y.Ma, R. J.Needs, F.Peng, C. J.Pickard, Y.Sun, Q.Wu. Hydrogen clathrate structures in rare earth hydrides at high pressures: Possible route to room-temperature superconductivity. Phys. Rev. Lett., 119, 107001(2017).
[47] F. F.Balakirev, L.Balicas, S. P.Besedin, A. P.Drozdov, M. I.Eremets, D. E.Graf, E.Greenberg, D. A.Knyazev, P. P.Kong, M. A.Kuzovnikov, V. S.Minkov, S.Mozaffari, V. B.Prakapenka, M.Tkacz. Superconductivity at 250 K in lanthanum hydride under high pressures. Nature, 569, 528(2019).
[48] M.Ahart, M.Baldini, Z. M.Geballe, R. J.Hemley, Y.Meng, A. K.Mishra, M.Somayazulu, V. V.Struzhkin. Evidence for superconductivity above 260 K in lanthanum superhydride at megabar pressures. Phys. Rev. Lett., 122, 027001(2019).
[49] N. W.Ashcroft, R. J.Hemley, R.Hoffmann, H.Liu, I. I.Naumov. Potential high-
[50] N.Dasenbrock-Gammon, R. P.Dias, K. V.Lawler, R.McBride, N.Meyers, A.Salamat, E.Snider, X.Wang, E.Zurek. Synthesis of yttrium superhydride superconductor with a transition temperature up to 262 K by catalytic hydrogenation at high pressures. Phys. Rev. Lett., 126, 117003(2021).
[51] W.Chen, T.Cui, D.Duan, X.Huang, X.Li, B.Liu, A. R.Oganov, D. V.Semenok, H.Xie, D.Zhou. Superconducting praseodymium superhydrides. Sci. Adv., 6, eaax6849(2020).
[52] H.Liu, J.Lv, Y.Ma, Y.Sun. Theory-orientated discovery of high-temperature superconductors in superhydrides stabilized under high pressure. Matter Radiat. Extremes, 5, 068101(2020).
[53] X.-J.Chen, A.Gavriliuk, E.Greenberg, C.Ji, B.Li, H.-k.Mao, V.Prakapenka, V.Struzhkin, I.Troyan. Superconductivity in La and Y hydrides: Remaining questions to experiment and theory. Matter Radiat. Extremes, 5, 028201(2020).
[54] C. W.Glass, N.Hansen, A. R.Oganov. USPEX—Evolutionary crystal structure prediction. Comput. Phys. Commun., 175, 713(2006).
[55] A. O.Lyakhov, A. R.Oganov, H. T.Stokes, Q.Zhu. New developments in evolutionary structure prediction algorithm USPEX. Comput. Phys. Commun., 184, 1172(2013).
[56] K.Tanaka, J. S.Tse, Y.Yao. Metastable high-pressure single-bonded phases of nitrogen predicted via genetic algorithm. Phys. Rev. B, 77, 052103(2008).
[57] J.Lv, Y.Ma, Y.Wang, L.Zhu. Crystal structure prediction via particle-swarm optimization. Phys. Rev. B, 82, 094116(2010).
[58] J.Lv, Y.Ma, Y.Wang, L.Zhu. CALYPSO: A method for crystal structure prediction. Comput. Phys. Commun., 183, 2063(2012).
[59] D. C.Lonie, E.Zurek. X
[60] K. J.Michel, C.Wolverton. Symmetry building Monte Carlo-based crystal structure prediction. Comput. Phys. Commun., 185, 1389(2014).
[61] H.Gao, C.Liu, J.Sun, H.-T.Wang, K.Xia, D.Xing, J.Yuan. A novel superhard tungsten nitride predicted by machine-learning accelerated crystal structure search. Sci. Bull., 63, 817(2018).
[62] R.Arita, F.Belli, R.Bianco, M.Calandra, I.Errea, J. A.Flores-Livas, T.Koretsune, F.Mauri, L.Monacelli, A.Sanna, T.Tadano. Quantum crystal structure in the 250-kelvin superconducting lanthanum hydride. Nature, 578, 66(2020).
[63] M.Calandra, I.Errea, Y.Li, H.Liu, Y.Ma, F.Mauri, R. J.Needs, J. R.Nelson, C. J.Pickard, Y.Zhang. Quantum hydrogen-bond symmetrization in the superconducting hydrogen sulfide system. Nature, 532, 81(2016).
[64] N.Bernstein, C. S.Hellberg, M. D.Johannes, I. I.Mazin, M. J.Mehl. What superconducts in sulfur hydrides under pressure and why. Phys. Rev. B, 91, 060511(2015).
[65] J.-H.Cho, J.Kim, K. W.Kim, L.Liu, C.Wang, S.Yi. Microscopic mechanism of room-temperature superconductivity in compressed LaH10. Phys. Rev. B, 99, 140501(2019).
[66] A. P.Drozdov, M.Einaga, M. I.Eremets, N.Hirao, T.Ishikawa, Y.Ohishi, M.Sakata, K.Shimizu, I. A.Troyan. Crystal structure of the superconducting phase of sulfur hydride. Nat. Phys., 12, 835(2016).
[67] Y.Ge, Y.Yao, F.Zhang. First-principles demonstration of superconductivity at 280 K in hydrogen sulfide with low phosphorus substitution. Phys. Rev. B, 93, 224513(2016).
[68] B. M.Klein, M. J.Mehl, D. A.Papaconstantopoulos, W. E.Pickett. Cubic H3S around 200 GPa: An atomic hydrogen superconductor stabilized by sulfur. Phys. Rev. B, 91, 184511(2015).
[69] T.Cui, D.Duan, X.Huang, D.Li, B.Liu, Y.Liu, Y.Ma, F.Tian, H.Yu. Pressure-induced decomposition of solid hydrogen sulfide. Phys. Rev. B, 91, 180502(2015).
[70] E. D.Bauer, N. J.Curro, E. A.Ekimov, N. N.Mel’nik, V. A.Sidorov, S. M.Stishov, J. D.Thompson. Superconductivity in diamond. Nature, 428, 542(2004).
[71] K.-W.Lee, W. E.Pickett. Superconductivity in boron-doped diamond. Phys. Rev. Lett., 93, 237003(2004).
[72] F.Chen, C.-W.Chu, A. M.Guloy, B.Lorenz, B.Lv, K.Sasmal, Y.-Y.Xue. Superconducting Fe-based compounds (A1−
[73] D. N.Argyriou, P. C.Canfield, T.Chatterji, E.Colombier, A. I.Goldman, T. C.Hansen, H. O.Jeschke, S. A. J.Kimber, A.Kreyssig, R. J.McQueeney, R.Valentí, J.Yan, F.Yokaichiya, Y.-Z.Zhang. Similarities between structural distortions under pressure and chemical doping in superconducting BaFe2As2. Nat. Mater., 8, 471(2009).
[75] T.Iitaka, Y.Ma, K.Tanaka, J. S.Tse, H.Wang. Superconductive sodalite-like clathrate calcium hydride at high pressures. Proc. Natl. Acad. Sci. U. S. A., 109, 6463(2012).
[76] J.-H.Cho, H.Jeon, C.Wang, S.Yi. Stability and bonding nature of clathrate H cages in a near-room-temperature superconductor LaH10. Phys. Rev. Mater., 5, 024801(2021).
[77] J.-H.Cho, S.Liu, C.Wang, S.Yi. Underlying mechanism of charge transfer in Li-doped MgH16 at high pressure. Phys. Rev. B, 102, 184509(2020).
[78] J.-H.Cho, C.Wang, S.Yi. Multiband nature of room-temperature superconductivity in LaH10 at high pressure. Phys. Rev. B, 101, 104506(2020).
[79] S. F.Elatresh, E. J.Nicol, T.Timusk. Optical properties of superconducting pressurized LaH10. Phys. Rev. B, 102, 024501(2020).
[80] P. H.Chang, M. J.Mehl, D. A.Papaconstantopoulos. High-temperature superconductivity in LaH10. Phys. Rev. B, 101, 060506(2020).
[81] J.-H.Cho, C.Wang, S.Yi. Pressure dependence of the superconducting transition temperature of compressed LaH10. Phys. Rev. B, 100, 060502(2019).
[82] A. P.Durajski, M.Kostrzewa, K. M.Szcz??niak, R.Szcz??niak. From LaH10 to room–temperature superconductors. Sci. Rep., 10, 1592(2020).
[83] X.-J.Chen, Y.-L.Hai, M.-J.Jiang, W.-J.Li, N.Lu, H.-L.Tian, X.-W.Yan, W.Yang, C.Zhang, G.-H.Zhong. Cage structure and near room-temperature superconductivity in TbH
[84] R.Ahuja, T.Bovornratanaraks, W.Luo, U.Pinsook, P.Tsuppayakorn-aek. Superconductivity of superhydride CeH10 under high pressure. Mater. Res. Express, 7, 086001(2020).
[85] H.-L.Chen, W.-C.Lu, D.Wang, J.Wu, Q.-J.Zang, H.Zhang. Theoretical study on UH4, UH8 and UH10 at high pressure. Phys. Lett. A, 383, 774(2019).
[86] Z.-w.Gu, C.-l.Liu, J.Liu, C.-w.Sun, F.-l.Tan, X.-h.Wang, P.Zhang, J.-h.Zhao, F.-w.Zheng. Hydrogen clathrate structures in uranium hydrides at high pressures. ACS Omega, 6, 3946(2021).
[87] V. Y.Fominski, A. G.Ivanova, A. G.Kvashnin, A. R.Oganov, V. M.Pudalov, A. V.Sadakov, D. V.Semenok, O. A.Sobolevskiy, V.Svitlyk, I. A.Troyan. Superconductivity at 161 K in thorium hydride ThH10: Synthesis and properties. Mater. Today, 33, 36(2020).
[88] X.Feng, G.Gao, H.Liu, H.Wang, J.Zhang. Compressed sodalite-like MgH6 as a potential high-temperature superconductor. RSC Adv., 5, 59292(2015).
[89] J.Hao, Y.Li, H.Liu, Y.Ma, J. S.Tse, Y.Wang. Pressure-stabilized superconductive yttrium hydrides. Sci. Rep., 5, 9948(2015).
[90] N. W.Ashcroft, R.Hoffmann, X.Ye, N.Zarifi, E.Zurek. High hydrides of scandium under pressure: Potential superconductors. J. Phys. Chem. C, 122, 6298(2018).
[91] R.Akashi, A.Bergara, R.Bianco, M.Calandra, I.Errea, A. G.Gavriliuk, E.Greenberg, A. G.Ivanova, A. G.Kvashnin, I. S.Lyubutin, F.Mauri, L.Monacelli, A. R.Oganov, V. B.Prakapenka, V. M.Pudalov, A. V.Sadakov, D. V.Semenok, O. A.Sobolevskiy, V. V.Struzhkin, I. A.Troyan. Anomalous high-temperature superconductivity in YH6. Adv. Mater., 33, 2006832(2021).
[92] T.Cui, D.Duan, D.Li, B.Liu, Z.Shao, H.Song, F.Tian, Y.Wang, X.Xiao, H.Xie. High-temperature superconductivity in ternary clathrate YCaH12 under high pressures. J. Phys.: Condens. Matter, 31, 245404(2019).
[93] A.Bergara, G.Gao, J.He, X.Liang, Y.Tian, L.Wang, B.Wen, Z.Zhao, X.-F.Zhou. Potential high-
[94] I. A.Kruglov, A. G.Kvashnin, A. R.Oganov, D. V.Semenok. Actinium hydrides AcH10, AcH12, and AcH16 as high-temperature conventional superconductors. J. Phys. Chem. Lett., 9, 1920(2018).
[95] H.Liu, K.Tanaka, J. S.Tse. Electron-phonon coupling mechanisms for hydrogen-rich metals at high pressure. Phys. Rev. B, 96, 100502(2017).
[96] T.Cui, D.Duan, X.Feng, S.Jiang, V. Z.Kresin, C. J.Pickard, S. A. T.Redfern, H.Song, H.Xie, Y.Yao, Z.Zhang. Hydrogen pentagraphenelike structure stabilized by hafnium: A high-temperature conventional superconductor. Phys. Rev. Lett., 125, 217001(2020).
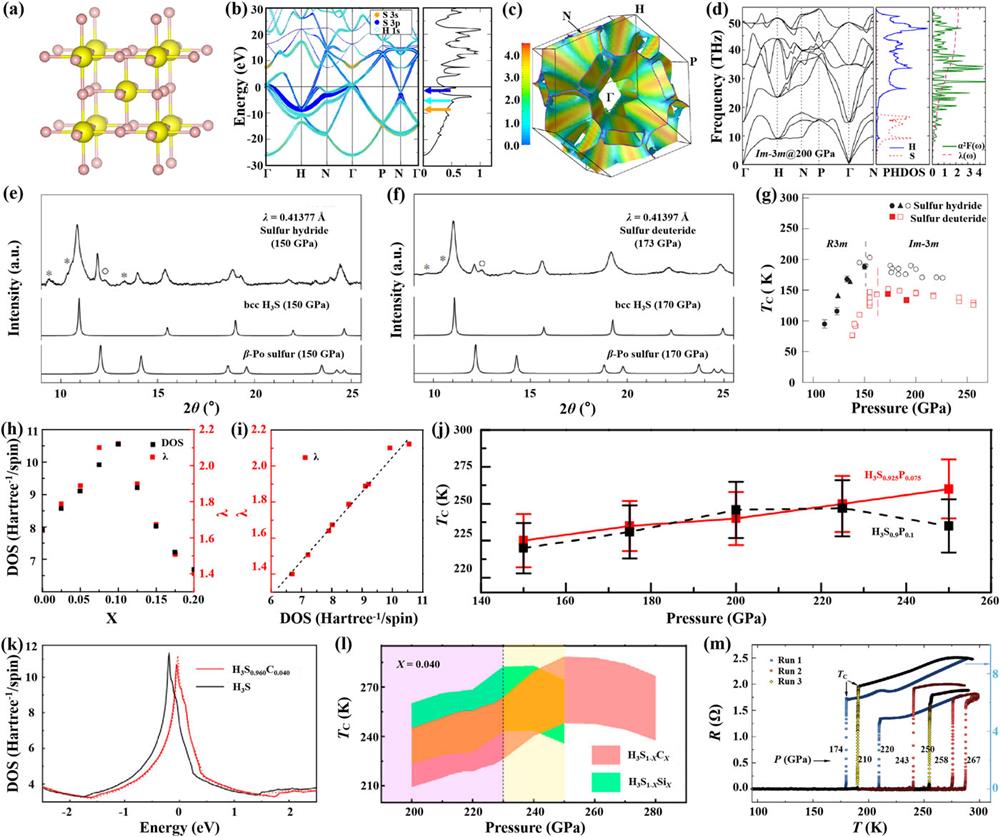
Set citation alerts for the article
Please enter your email address