
- Matter and Radiation at Extremes
- Vol. 5, Issue 2, 26402 (2020)
Abstract
I. INTRODUCTION
Thermonuclear fusion burst onto the scene in the early 1950s with almost unlimited explosive power, and, ever since, thermonuclear fusion has been thought of as a potentially unlimited supply of energy: fission became useful as an energy source almost overnight, so why not fusion? A linear Z-pinch was an early attempt to create a fusion-relevant plasma in the laboratory. The microsecond-long pulsed power available at the time did indeed produce neutrons that proved a nuclear reaction. Fusion energy seemed right around the corner, and the world’s nuclear powers tried to make this happen by themselves.
As is now well known, instabilities in the linear Z-pinch give some ions enough energy to overcome the multi-keV Coulomb barrier between two deuterium (D) or tritium (T) nuclei. A few energetic ions can then participate in an exothermic nuclear reaction, but the resulting energy is much less than the energy that the vast majority of these energetic ions lose to the plasma. Thermonuclear fusion demands constant recycling of the energy so that the DT mixture remains full of energetic ions, that is, it is a DT plasma.
Once the nuclear powers with thermonuclear energy programs realized that they could not achieve quick success on their own, they decided to share their research in what is now known as magnetic fusion energy, with ever-increasing cooperation between the actors. The hope is to create a stable DT plasma with thermonuclear parameters as in the ITER tokamak, a joint project between many nations that should become operational three-quarters of a century after the initial attempts with the Z-pinch. Thermonuclear fusion research with the available pulsed power continued in the dense plasma focus; the practitioners hoped that instabilities could somehow be dealt with: either suppressed or otherwise used to good advantage.
An alternative, inertial confinement fusion (ICF), joined the party in the 1970s. ICF tries to achieve thermonuclear conditions by compressing a small DT sphere from all sides with a short pulse of power, from x-rays or otherwise: the aim is for inertia to keep the DT plasma dense enough for sufficiently long to produce appreciable energy. A quite recent development is magnetized liner inertial fusion (MagLIF), which attempts to compress a preheated plasma inside an imploding Z-pinch shell, wherein the magnetic field keeps heat loss to the outside within limits.
What Z-pinches do depends ultimately on the available pulsed power. Typically, this is a short (∼100 ns or less), high-voltage (∼0.1–1 MV or higher), and high-current (∼0.1–10 MA and higher) electrical discharge. In the UK, the technology came largely from J. C (Charlie) Martin
Figure 1.Advances in pulsed power illustrated with the machines at Sandia National Laboratory (SNL).
These γ-ray pulses are far off the right side in
Figure 2.Progress in the radiation per pulse achievable with Z or NIF from 2012 to 2017. A future fusion facility would produce substantial radiation in the difficult region around ∼60 keV.
An electron in a space-charge-limited diode converts its energy into radiation only once, with an efficiency η ∼ V2–3 or so, which makes it impossible to get powerful enough x-ray pulses below ∼100 keV with a vacuum diode. Instead, the electrons must continually regain their energy so that they can make radiation multiple times; that is, there must be a plasma. The plasma can come from a powerful laser (NIF in
Very little material is required to suppress space change: the charge Q = −Iτ = −0.1 C carried by an I ∼ 1 MA pulse over τ ∼ 100 ns is the same as ∼1 µg of any material has in its electrons. The art of the Z-pinch is, then, to have the discharge start through some material that connects the electrodes electrically, in some clever way that makes the best use of the electrical energy available in the pulse to optimize the intended output. This is very difficult when the discharge can have only a small amount of material: it could be blown off an insulator, injected as a gas, or put in as thin wires.
Figure 3.Initial state of Z-pinches that are subjects at the DZP conference.
All of these initial conditions eventually end up with the diode material becoming a hot plasma. Heating is in part instantaneous Ohmic heating VI, where V = IR (and defines the resistance R). Often the larger power source is conversion of kinetic energy stored in an implosion into heat; the voltage is then inductive (V = I dL/dt) and corresponds to the power needed to fill the diode with magnetic energy.
Naively, the highest density, and most powerful radiation, should occur along an axis of cylindrical symmetry, but this is not always the case. Implosions are highly unstable: with a nominally ideal, cylindrically symmetric initial condition, a Z-pinch may radiate softer x-rays from an ideal plasma cylinder as in
Figure 4.(a) An idealized Z-pinch in early modeling. (b) On a microscale, the plasma is made up of electrons and different kinds of ions that interact with photons.
Radiating Z-pinches are tightly bound up with pulsed power. Besides the generators at SNL mentioned in
For thermonuclear fusion, the temperature T in a DT plasma must reach close to 10 keV, the density n must be large enough, and it must remain so for enough time τ: one (Lawson-like) parameter is nTτ (as in
The shaded areas in
In between the plasma radiators on the left and bremsstrahlung on the right of
In the USA, the Department of Energy (DOE) funds research aimed at thermonuclear fusion, and much research on pulsed radiation as well. The DOD deals with x-rays only, for which it sponsors research at government laboratories such as NRL, at private companies like PI (or L3Harris), and at universities. On this softer Z-pinch side of
The vagaries in Z-pinch research are typical of applied work: irrespective of whether some stated goal is reached or abandoned, the focus of interest can shift, as is reflected in
SNL’s present ICF direction is magnetized inertial fusion (MagLIF),
Building and operating large pulsed power generators is expensive and time-consuming, and so is research with them. Hence, many smaller pulsed power generators that can still create relevant plasmas are important in Z-pinch research, and are quite productive: the diagnostics from plasmas made by smaller machines are just as challenging to interpret as those from the massive facilities. Already mentioned are the ∼1 MA generators at IC in the UK and at Cornell and the University of Michigan in the USA; similar research goes on at the University of California, San Diego (UCSD) in the USA, in Russia, China, Israel, and elsewhere. Still smaller machines make interesting plasmas as well, in, for example, Chile, Singapore, and Japan. Besides suggesting intellectual challenges, the larger laboratories motivate research at the smaller sites by personal contacts among students and scientists, and through funding for aspiring students and their eventual employment.
This paper reinterprets the talk that I gave at the 11th DZP conference. It is a personal view of the field in which I was active early on and of which I later became a sympathetic observer from the outside. I apologize upfront for any misconceptions I may have, for selecting my favorite topics over yours, and for freely taking information I deemed reliable from the web: distressingly many pitches for fusion are misleading nonsense.
II. WHERE DOES THE DZP CONFERENCE FIT IN?
When a small, specialized, and growing field of research exceeds a certain size, it deserves a small, specialized conference for practitioners in the field to get together, learn about what is going on elsewhere, comment on each other’s work, and get to know each other so that off-site communication becomes less of a hurdle. They complement the larger meetings with their many parallel sessions, where a smaller field can get drowned out. Z-pinches for radiation and fusion remain topics at the annual plasma conferences such as the APS-DPP (Division of Plasma Physics) conference and its analogues elsewhere, at the IEEE-ICOPS (Plasma Science), and the IEEE-PPS (Pulsed Power).
Z-pinches reached this point in the mid 1980s, when pulsed radiation production with Z-pinches blossomed in the open. The DZP conference was modeled on the BEAMS conference, which dealt with pulsed-power-driven particle beams and their applications, including ICF and radiation from vacuum diodes as on the right side of
As the interest in a technical field changes and possibly decreases, its specialized conference may have to adapt: BEAMS did this by combining with DZP in 2002 in Albuquerque, NM, USA, and most recently in 2018 with the East Asia Pulsed Power Conference in Changsha, China. The DZP may well have to adapt in a similar way, since pulsed radiation production with Z-pinches is no longer so compelling, and funding for the topic may decrease.
A. The first DZP1984: “Dense Z-Pinches for fusion”
NRL’s John Sethian organized the first International Conference on Dense Z-Pinches, DZP1984, in Alexandria, VA, USA, with a clear purpose in mind. In the introduction to the proceedings, he states, essentially:
… this meeting is singularly dedicated towards attempts to develop the Z-pinch into a source of thermonuclear energy. At first glance a renewed attempt with Z-pinches seems futile: dense Z-pinches have generated 14 MeV neutrons since the earliest days of fusion. However, recent advances in pulsed power suggest that Z-pinch fusion is still in its infancy. Fusion reactor concepts based on the elegant and simple Z-pinch seem much more attractive than magnetic confinement or other schemes.
Indeed, one such reactor concept was presented at that first DZP.
The High Density Z-Pinch (HDZP) program at Los Alamos National Laboratory (LANL) is an example of the thinking at the time. It envisions a stable DT pinch as in
As it turns out, in deuterium, pinches do not collapse like this, but collapse-like micropinches can occur when the atomic number Z is larger and atomic transitions make the radiation much stronger than bremsstrahlung.
This first DZP helped NRL to explore Z-pinch fusion, first with a pinch that was thought to remain stable inside a capillary, and eventually with a cryogenic deuterium wire. The resulting pinches indeed seemed stable and produced neutrons, but they turned out not to be thermonuclear: the deuterium core remained solid and cold while a low-density, low-resistance plasma outside carried the current. Plasmas blown off from wire or metal when it carries a high surface current remain a problem today, on the one hand because they are not easy to model properly and on the other because they are difficult to deal with experimentally.
The NRL machine vanished, but the LANL machine resurfaced as Zebra at the University of Nevada, Reno (UNR), where it was very productive (as in
Despite the failure to produce fusion energy with a Z-pinch that started off DZPs, thermonuclear fusion research keeps going in part because of its enormous payoff, “The Final Solution to the World’s Energy Problem.” Its lack of success so far may disappoint, but is not so bad: overcoming obstacles needs plasma physicists who remain gainfully employed.
B. The second DZP1989: Add x-rays and Soviet scientists
In the USA in the 1980s, President Reagan’s Star Wars initiative imagined military applications for intense beams of protons, electrons, and all kinds of photons from microwaves to hard x-rays. All needed similar pulsed power, but different front ends. By then, Z-pinches had shown ever-larger radiation outputs (but still much lower than in
During the Cold War, the USA and the Soviet Union (USSR) had been in respectful competition on such research, with occasional technical exchange visits by prominent scientists, including Norman Rostoker to the USSR and Leonid Rudakov to the USA. Perestroika in the late 1980s and American money made it possible for seven Soviet scientists to attend the second DZP conference.
This conference was very successful. Attendance was about one-third non-US, and hence truly international. The attendees decided to continue with DZPs on a quadrennial schedule, rotating between the locations where most Z-pinch research occurred: the USA, the UK, Continental Europe, and the USSR. In analogy to the BEAMS conference, a Governing Board would consist of previous Conference Chairs. The scientific emphasis would tilt to the front end, where a small change can make a large difference. Some are sketched in
C. The third DZP1993 and later ones
Malcolm Haines organized the third conference in London, UK. As before, Continental Europe, the UK, and the USA were well represented. Many scientists with backgrounds in the USSR came too, some now from Russia and many with affiliations elsewhere (including S. I. Braginskiǐ). He and R. S. Pease, both of IPB fame, inaugurated IC’s newly built MAGPIE generator. In retrospect, this conference may have been a high point for DZPs: defense-relevant research had not yet decreased and visas for scientists to travel to the USA had not yet become so uncertain.
The effects of the world political climate continued to be seen at the DZP. Instead of the next meeting being in the USSR, DZP1997 moved to Vancouver, Canada so that the conference remained visibly international. Fewer attendees with Soviet pedigrees came from Russia than from elsewhere, since many had joined foreign institutions, where some were already key figures in Z-pinch research. Leonid Rudakov, now in the USA, alerted the attendees to 1800-vintage pulsed power,
One prominent attendee was R. S. Pease, who by now had retired as Director of the UK’s thermonuclear fusion research efforts. In his keynote address, he confessed to being disappointed with the progress in fusion: in about 1975, he had expected to have thermonuclear energy by now. Another was Lu Ming-fang (Chinese Academy of Sciences), the first scientist from China to attend a DZP, who discussed work on a small plasma focus.
In the decade after the end of the Cold War, sometime in the early 1990s, the military’s interest in particle beams and x-ray pulses dwindled. In contrast, success with ICF-relevant quasi-blackbody radiation from multiwire tungsten Z-pinches at SNL had spurred an upgrade of Z to ZR. To match this upgrade, the next DZP conference was delayed by one year and combined with BEAMS. This joint conference had 12 attendees from China: three of them organized the present DZP (J.-J. Deng, N. Ding, and C. Ning). Their papers now included research on large pulsed power generators built in China.
On the technical level, each subsequent conference testified to the increased power of computers, from the quality of the visuals in the presentations to the increasing physics content in numerical modeling. Likewise, diagnostics became ever more powerful. Progress in the field is best seen from the reviews that appear periodically, some in connection with a DZP and others with other conferences. The most extensive, that by Haines,
One thing that is remarkable is the continuously increasing prominence of Chinese scientists at the DZP. One exception is DZP2008, when US visa problems prevented their attendance. Bureaucratic problems happen in reverse as well: US Government rules prevented US Government scientists coming to China for this 11th DZP, much to their chagrin.
III. PROGRESS IN THEORY
This personal account does not intend to review the technical progress, which is better done by the papers in
Title or topic | Reference |
---|---|
X-rays from Z-pinches… | |
Plasma points and radiative collapse… | |
Scientific status of plasma focus research | |
Physics of High-Density Z-Pinch Plasmas | |
The physics of fast Z pinches | |
The past, present, and future of Z pinches | |
A review of the dense Z-pinch | |
Magnetically driven implosions for ICF at SNL | |
…Z-pinch as an X-ray and neutron source… | |
Characterizing the plasmas of dense Z-pinches | |
X-pinch I, II, III | |
…wire array Z-pinch and dynamic hohlraum… |
Table 1. A selection of Z-pinch-relevant summaries.
The Z-pinch cartoon on the left in
Adding power equilibrium gives another analytical relation, the Pease–Braginskiǐ currentin the form from Ref.
At the start of an electrical pulse, the pinch in
Unlike the featureless fluid plasma in
For such higher atomic number plasmas, atomic radiation is more intense than bremsstrahlung. One consequence is the dimensionless factor in Eq.
The present approach is largely numerical. The ever-increasing capabilities of computers allow the codes to include more phenomena and treat these more accurately; ever-better computer graphics and even movies give seductive images that range from misleading to insightful.
Figure 5.Gorgon simulation of an 8-wire Z-pinch,
The x-rays emitted by Z-pinches are usually estimated in collisional radiative equilibrium (CRE), where Maxwellian electrons are locally in thermal equilibrium with the different ion species as these radiate away the plasma’s energy, and the radiation itself spreads the energy throughout the plasma, nonlocally. The atomic physics is now well enough known for a code on the web (FLYCHK
The approximations can be done in many ways that are compared regularly in the biennial Non-Local Thermodynamic Equilibrium (NLTE) workshops.
Figure 6.A temperature-sensitive line ratio in Ne computed at the NLTE workshop.
At the conference, I illustrated one approximation to CRE with my favorite example: non-Maxwellian electrons. Every x-ray that leaves the plasma implies at least one inelastic collision wherein an energetic electron loses the corresponding energy. Getting that energy back takes multiple Coulomb collisions. With inelastic collisions taken into account, in one computation,
Exploring plasma physics with abridged models remains useful: a particle-in-cell (PIC) computation at the conference
Despite the approximations in the models, radiation-MHD computations of Z-pinches do produce x-ray spectra that may result in credible numbers for the plasma parameters. Despite the inevitable shortcomings, the modeling effort itself is essential for Z-pinch research: how else to give our sponsors the numbers they want, and to satisfy our desire to know what goes on in the plasma from ever-improving diagnostics?
IV. PROGRESS IN MEASUREMENTS
In reality, an imploded plasma is not a uniform cylinder as in
Figure 7.Densitograms of the same Al wire plasma obtained with two laser wavelengths, and one corresponding interferogram. Reprinted with permission from Ivanov
Pulsed lasers can give spatially and time-resolved images of the current density as described recently in this journal,
I could have picked any number of excellent x-ray spectroscopy diagnostics to illustrate what can be done with x-rays. The one I showed was the time-resolved Doppler shift of an Mg impurity’s He-α line in an Al wire implosion, which finds the implosion velocity of the material that makes the x-rays.
V. WHERE MIGHT Z-PINCH RESEARCH GO?
In academia, scientific interest in a particular topic may well keep an individual researcher occupied for their entire career, but in an applied and policy-sensitive field such as radiative Z-pinches, the direction and most importantly the funding come from some government agency. Thermonuclear fusion is sometimes supported by rich individuals or private organizations, investors who accept that most research does not pay off but hope that they are the lucky ones to grab a “unicorn,” the single exception that makes up for all the failures. While unusual in a scientific paper, I found it appropriate to comment on these matters at a DZP conference.
I briefly mentioned the multiwire Z-pinch identified with Tom Sanford and others at SNL. In papers starting with Ref.
Changes in research direction may have less happy outcomes. At the meeting, I mentioned one in radiation and one in fusion.
A. The saga of decade
Nuclear explosions result in giant plasmas that radiate short, powerful x-ray pulses over the entire photon spectrum up to many MeV. Governments that worry about nuclear weapons would want to have laboratory sources that are similar. When such concerns recede, research in this direction may then lose its urgency, as happened with Z-pinches. A painful transition period with ever-decreasing funding then leaves all kinds of interesting science abandoned and equipment repurposed.
When MeV-type x-ray pulses seemed important in the USA in the 1960s and 1970s, a 10 MV pulsed power machine with 1 MA peak current such as Aurora could be built. Its ∼10 MJ capacitor bank is shown in
Figure 8.Three fateful pulsed power generators: (a) Aurora; (b) Decade-Quad in its Z-pinch configuration; (c) the Decade module that survives as Charger-1. For a scale, identify the stairs in (a) and (b).
A few decades later, the lesson had been forgotten, as is illustrated by
The eventual choice became to first try for 10× more bremsstrahlung, on the right side in
While the original ∼100 MA peak current seemed infeasible, with a high-current machine like Decade-Quad in the right place already, the most affordable path toward the highest Ip seemed to be to add a Z-pinch option.
Decade-Quad’s Z-pinch encountered the usual technical setbacks, in part resulting from a nontechnical issue: Decade-Quad’s location lacked technical people with the attitude needed for pulsed power and scientific staff with the expertise. Eventually, Decade-Quad’s Z-pinch did achieve its promised output, but by that time the program had already been canceled because the need for a more powerful Z-pinch had receded.
As with Phoenix, some of the hardware lives on. One-quarter of Decade-Quad is now Charger-1, seen in
B. Thermonuclear fusion: Still at the end of the rainbow
Hope springs eternal, and paper is patient.
Figure 9.Neutron yields per centimeter in D2 pinches: achieved and extrapolated. After Ref.
In my talk, I expressed my admiration for those who manage to obtain funding for speculative attempts to develop thermonuclear fusion commercially, especially when they sell an explosion to do it when experience so far shows how difficult that would be. Right now, the record is ∼0.05 MJ in thermonuclear energy, with a ∼10 MJ discharge. In a high-value application such as x-ray simulation, which demands months of preparation before and months of analysis after each experiment, single-shot fusion is completely acceptable despite its expense.
It is perfectly possible to get useful energy from repeated explosions, but this works best when the hardware survives: in a car, each cylinder produces up to 1 kJ per explosion, at 100 Hz. For a 100 MW Z-pinch fusion that makes ∼100 MJ thermonuclear energy per pulse each second, with a comparable amount to drive the explosion, hardware cannot survive: one tank of gasoline has ∼1 GJ in energy. Admirable efforts
Perhaps for reasons similar to the above, the US Government funds thermonuclear energy only for those efforts that have some additional payoff.
Figure 10.Triple-product fusion parameter
Achieving a megajoule-class thermonuclear explosion by ICF, on a pulsed power machine with a Z-pinch or with a laser, is a worthy and also a reasonably achievable goal: NIF has reportedly reached 30% of the threshold for ignition.
Hopefully, governments in the USA and elsewhere will continue to support research on high-energy-density plasmas, including those in Z-pinches. The scientific and technical challenges are more than sufficient to keep a scientist interested and to attract students to plasma physics. The desire to fill the bucket in
References
[1] General background on this topic can be found via a web search on the term “history of fusion”.
[2] M. Christianson, A. H. Gunther, T. H. Martin. J C Martin on Pulsed Power(1996).
[4] S. A. Slutz et al. Phys. Plasmas, 17, 056303(2010).
[5] S. M. Miller et al. Laser gate experiment for increasing preheat energy coupling efficiency in magnetized liner inertial fusion (MagLIF). Matter Radiat. Extremes(2019).
[6] J. Woolstrum et al. Validation of perseus and convergence ratio effects on
[7] S. Bland et al. New insights into the pulsed power driven explosion of underwater wires and wire arrays. Matter Radiat. Extremes(2019).
[8] S. Lebedev et al. Z-pinch driven laboratory astrophysics experiments at imperial college. Matter Radiat. Extremes(2019).
[10] https://en.wikipedia.org/wiki/Large_electrostatic_generator_(Teylers)
[12] Pulsed power’s potential for military use has a long history. Around 1800 the French Emperor Napoleon came to Teyler’s museum to look this capacitor bank for its military potential. It was built in The Netherlands around 1790, by an English craftsman working abroad; Charlie Martin did something similar but in the US. The relative cost of the largest machines (∼1 $/J) remained roughly similar as well.
[13] M. G. Haines. A review of the dense Z-pinch. Plasma Phys. Controlled Fusion, 53, 093001(2011).
[16] S. Cordaro et al. High voltage coaxial vacuum gap breakdown for pulsed power liners. Matter Radiat. Extremes(2019).
[18] J. Chittenden et al.
[22] A. Safronova et al. Overview of x-ray time-gated spectroscopy and imaging of 1 Ma wire array
[23] H. Chung, C. Fontes, S. Hansen, Yu. Ralchenko, H. Scott, E. Stambulchik(2019).
[25] C. Ning et al. Numerical invesigation into the
[32] K. Ware et al(1992).
[34] D. Klir et al. Deuterium gas-puff
[35] W. Zou et al. Progress and outlook of pulsed power driver on the road to fusion. Matter Radiat. Extremes(2019).
[36] T. Clayson et al. M3: A new pulsed power machine dedicated to inertial confinement fusion experiments. Matter Radiat. Extremes(2019).
[38] H. C. Andersen. The Emperor’s New Clothes(1837).
[39] P. Patel et al. Progress towards achieving ignition on the National Ignition Facility.
[42] A. Bernard et al. Scientific status of plasma focus research. J. Moscow Phys. Soc., 8, 93(1998).
[43] M. A. Liberman et al. Physics of High-Density Z-Pinch Plasmas(1999).
[45] M. G. Haines et al. The past, present, and future of
[46] M. Cuneo et al. Magnetically driven implosions for ICF at SNL. IEEE Trans. Plasma Sci., 40, 3222(2012).
[48] S. A. Pikuz, S. A. Pikuz, S. A. Pikuz et al. X-Pinch. III. Fiz. Plazmy, 42, 234(2016).
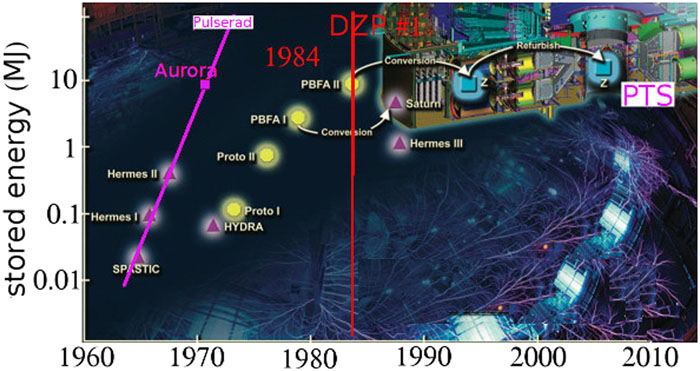
Set citation alerts for the article
Please enter your email address