
- Chinese Optics Letters
- Vol. 19, Issue 6, 060006 (2021)
Abstract
1. Introduction
Lithium niobate on insulator (LNOI) is emerging as a promising platform for integrated photonics thanks to its large electric-optic coefficient, strong second-order optical nonlinearity, low absorption loss, and wide transparent window (from 400 nm to 5 µm)[
Despite these advances, efficient coupling between a lithium niobate (LN) chip and optical fiber is still a major challenge, mainly due to the large mode mismatch between the fiber mode (diameter of 10.4 µm) and waveguide mode[
Recently, several one-dimensional (1D) GCs have been demonstrated on the LNOI platform[
Sign up for Chinese Optics Letters TOC. Get the latest issue of Chinese Optics Letters delivered right to you!Sign up now
The two-dimensional (2D) GC, an important building block in photonic integrated circuits (PICs) to overcome polarization-dependence issues, enables coupling between the fiber and waveguide modes (TE polarization), regardless of the fiber mode polarization. Many efforts have been devoted to improving the performance of 2D GCs on the silicon on insulator (SOI) platform, including integrating a backside metal mirror[
In this Letter, we demonstrate the first, to the best of our knowledge, 2D GC on the
2. Simulation and Design
The schematic structure of the proposed LN 2D GC is shown in Fig. 1, which is fabricated on x-cut LNOI wafers for the sake of serving LN modulators, which are mainly fabricated on x-cut LNOI wafers, benefiting from the strongest EO coefficient
Figure 1.(a) Schematic structure of x-cut LN 2D GCs. The angle between axis Z, Y (direction of grating) and optical axis z of LN material is β = 45°.The angle φ represents the polarization state of the fiber mode. (b) Cross-sectional view of the LN 2D GC.
Three-dimensional (3D) finite-difference time-domain (FDTD) method is utilized for the simulation. The simulation volume is
Firstly, we did a parameter sweep for E, P, and R to tune the central wavelength target on 1550 nm. In this step, the thickness of the BOX layer is fixed to
Figure 2.(a) Coupling efficiency of the 2D GC for different structure parameters, when HLN = 360 nm, Hb = 2 µm, θ = 10°, and α = 60°. (b) The dependence of coupling efficiency and etching depth for 1D and 2D GCs at a wavelength of 1550 nm. (c) The coupling efficiency as a function of BOX thickness Hb at 1550 nm, with the parameters E = 180 nm, P = 982 nm, and R = 400 nm for the 2D GC, and E = 180 nm, P = 1.02 µm, and no = 2.21, with a duty cycle of 0.38 for uniform 1D GCs. (d) Coupling efficiency of 1D and 2D GCs with a gold mirror when Hb = 2 µm.
Secondly, we calculated the angle between two LN waveguides using a large simulation volume of
Figure 3.Propagation of the coupled mode in the LN slab waveguide.
To investigate the performance sensitivity of 2D GCs to the input polarization state, we calculate the coupling efficiency of
Figure 4.(a) Coupling efficiency of CEZ, CEY, and CET as a function of the polarization angle φ. (b) Coupling spectra of CEZ, CEY, and CET when φ = 75°. (c) Relationship between the central wavelength λc and polarization angle φ.
Figure 5.Impact of the variation of (a) etching depth ΔE, (b) grating pitch ΔP, (c) hole radius ΔR, and (d) sidewall angle Δα.
3. Fabrication and Measurement
The proposed devices were fabricated on a commercial 360 nm thick
Figure 6.(a) SEM image of LN 2D GCs. The inset shows the zoom-in picture of grating cells. (b) Optical image of the LN 2D GCs. (c) Experimental set-up scheme: TL, tunable laser; PC, polarization controller; PM, power meter.
Figure 7(a) shows the measured spectra of the fabricated 1D and 2D GCs. The peak coupling efficiency of the 1D GC is about
Figure 7.(a) Measured and calculated coupling efficiencies for the LN 1D GC and 2D GC. (b) Measured coupling efficiencies of the LN 2D GC with different hole radii.
4. Conclusion
In summary, we have demonstrated the first 2D GC on the LNOI platform. The measured coupling efficiency is
References
[1] A. Boes, B. Corcoran, L. Chang, J. Bowers, A. Mitchell. Status and potential of lithium niobate on insulator (LNOI) for photonic integrated circuits. Laser Photon. Rev., 12, 1700256(2018).
[2] L. Cai, A. Mahmoud, G. Piazza. Low-loss waveguides on Y-cut thin film lithium niobate: towards acousto-optic applications. Opt. Express, 27, 9794(2019).
[3] M. Zhang, C. Wang, R. Cheng, A. Shams-Ansari, M. Lončar. Monolithic ultra-high-Q lithium niobate microring resonator. Optica, 4, 1536(2017).
[4] Y. Liu, X. Huang, Z. Li, Y. Kuang, H. Guan, Q. Wei, Z. Fan, Z. Li. TE/TM-pass polarizers based on lateral leakage in a thin film lithium niobate–silicon nitride hybrid platform. Opt. Lett., 45, 4915(2020).
[5] T. Ding, Y. Zheng, X. Chen. On-chip solc-type polarization control and wavelength filtering utilizing periodically poled lithium niobate on insulator ridge waveguide. J. Lightwave Technol., 37, 1296(2019).
[6] J.-Y. Chen, Z.-H. Ma, Y. M. Sua, Z. Li, C. Tang, Y.-P. Huang. Ultra-efficient frequency conversion in quasi-phase-matched lithium niobate microrings. Optica, 6, 1244(2019).
[7] D. Pohl, M. R. Escalé, M. Madi, F. Kaufmann, P. Brotzer, A. Sergeyev, B. Guldimann, P. Giaccari, E. Alberti, U. Meier, R. Grange. An integrated broadband spectrometer on thin-film lithium niobate. Nat. Photon., 14, 24(2020).
[8] L. Cai, A. Mahmoud, M. Khan, M. Mahmoud, T. Mukherjee, J. Bain, G. Piazza. Acousto-optical modulation of thin film lithium niobate waveguide devices. Photon. Res., 7, 1003(2019).
[9] M. Xu, M. He, H. Zhang, J. Jian, Y. Pan, X. Liu, L. Chen, X. Meng, H. Chen, Z. Li, X. Xiao, S. Yu, S. Yu, X. Cai. High-performance coherent optical modulators based on thin-film lithium niobate platform. Nat. Commun., 11, 3911(2020).
[10] M. He, M. Xu, Y. Ren, J. Jian, Z. Ruan, Y. Xu, S. Gao, S. Sun, X. Wen, L. Zhou, L. Liu, C. Guo, H. Chen, S. Yu, L. Liu, X. Cai. High-performance hybrid silicon and lithium niobate Mach–Zehnder modulators for 100 Gbit s−1 and beyond. Nat. Photon., 13, 359(2019).
[11] M. Zhang, B. Buscaino, C. Wang, A. Shams-Ansari, C. Reimer, R. Zhu, J. M. Kahn, M. Lončar. Broadband electro-optic frequency comb generation in a lithium niobate microring resonator. Nature, 568, 373(2019).
[12] Y. Qi, Y. Li. Integrated lithium niobate photonics. Nanophotonics, 9, 1287(2020).
[13] Z. Chen, Y. Ning, Y. Xun. Chirped and apodized grating couplers on lithium niobate thin film. Opt. Mater., 10, 2513(2020).
[14] J. Jian, P. Xu, H. Chen, M. He, Z. Wu, L. Zhou, L. Liu, C. Yang, S. Yu. High-efficiency hybrid amorphous silicon grating couplers for sub-micron-sized lithium niobate waveguides. Opt. Express, 26, 29651(2018).
[15] A. Kar, M. Bahadori, S. Gong, L. L. Goddard. Realization of alignment-tolerant grating couplers for z-cut thin-film lithium niobate. Opt. Express, 27, 15856(2019).
[16] I. Krasnokutska, R. J. Chapman, J.-L. J. Tambasco, A. Peruzzo. High coupling efficiency grating couplers on lithium niobate on insulator. Opt. Express, 27, 17681(2019).
[17] Y. Liu, X. Huang, Z. Li, H. Guan, Q. Wei, Z. Fan, W. Han, Z. Li. Efficient grating couplers on a thin film lithium niobate–silicon rich nitride hybrid platform. Opt. Lett., 45, 6847(2020).
[18] X. Ma, C. Zhuang, R. Zeng, J. J. Coleman, W. Zhou. Polarization-independent one-dimensional grating coupler design on hybrid silicon/LNOI platform. Opt. Express, 28, 17113(2020).
[19] M. S. Nisar, X. Zhao, A. Pan, S. Yuan, J. Xia. Grating coupler for an on-chip lithium niobate ridge waveguide. IEEE Photon. J., 9, 6600208(2016).
[20] Z. Ruan, J. Hu, Y. Xue, J. Liu, B. Chen, J. Wang, K. Chen, P. Chen, L. Liu. Metal based grating coupler on a thin-film lithium niobate waveguide. Opt. Express, 28, 35615(2020).
[21] K. Shuting, R. Zhang, Z. Hao, J. Di, F. Gao, F. Bo, G. Zhang, J. Xu. High-efficiency chirped grating couplers on lithium niobate on insulator. Opt. Lett., 45, 6651(2020).
[22] Z. Chen, Y. Wang, Y. Jiang, R. Kong, H. Hu. Grating coupler on single-crystal lithium niobate thin film. Opt. Mater., 72, 136(2017).
[23] I. Krasnokutska, J.-L. J. Tambasco, A. Peruzzo. Nanostructuring of LNOI for efficient edge coupling. Opt. Express, 27, 16578(2019).
[24] L. He, M. Zhang, A. Shams-Ansari, R. Zhu, C. Wang, L. Marko. Low-loss fiber-to-chip interface for lithium niobate photonic integrated circuits. Opt. Lett., 44, 2314(2019).
[25] Y. Luo, Z. Nong, S. Gao, H. Huang, Y. Zhu, L. Liu, L. Zhou, J. Xu, L. Liu, S. Yu, X. Cai. Low-loss two-dimensional silicon photonic grating coupler with a backside metal mirror. Opt. Lett., 43, 474(2018).
[26] B. Chen, X. Zhang, J. Hu, Y. Zhu, X. Cai, P. Chen, L. Liu. Two-dimensional grating coupler on silicon with a high coupling efficiency and a low polarization-dependent loss. Opt. Express, 28, 4001(2020).
[27] R. Halir, D. Vermeulen, G. Roelkens. Reducing polarization-dependent loss of silicon-on-insulator fiber to chip grating couplers. IEEE Photon. Technol. Lett., 22, 389(2010).
[28] W. Bogaerts, D. Taillaert, P. Dumon, D. Van Thourhout, R. Baets, E. Pluk. A polarization-diversity wavelength duplexer circuit in silicon-on-insulator photonic wires. Opt. Express, 15, 1567(2007).
[29] D. Bunandar, A. Lentine, C. Lee, H. Cai, C. M. Long, N. Boynton, N. Martinez, C. DeRose, C. Chen, M. Grein, T. Douglas, A. Starbuck, A. Pomerene, S. Hamilton, F. N. C. Wong, R. Camacho, P. Davids, J. Urayama, D. Englund. Metropolitan quantum key distribution with silicon photonics. Phys. Rev. X, 8, 021009(2018).
[30] L. Carroll, D. Gerace, I. Cristiani, S. Menezo, L. C. Andreani. Broad parameter optimization of polarization-diversity 2D grating couplers for silicon photonics. Opt. Express, 21, 21556(2013).
[31] Y. Wang, W. Shi, X. Wang, Z. Lu, M. Caverley, R. Bojko, L. Chrostowski, N. A. F. Jaeger. Design of broadband subwavelength grating couplers with low back reflection. Opt. Lett., 40, 4647(2015).
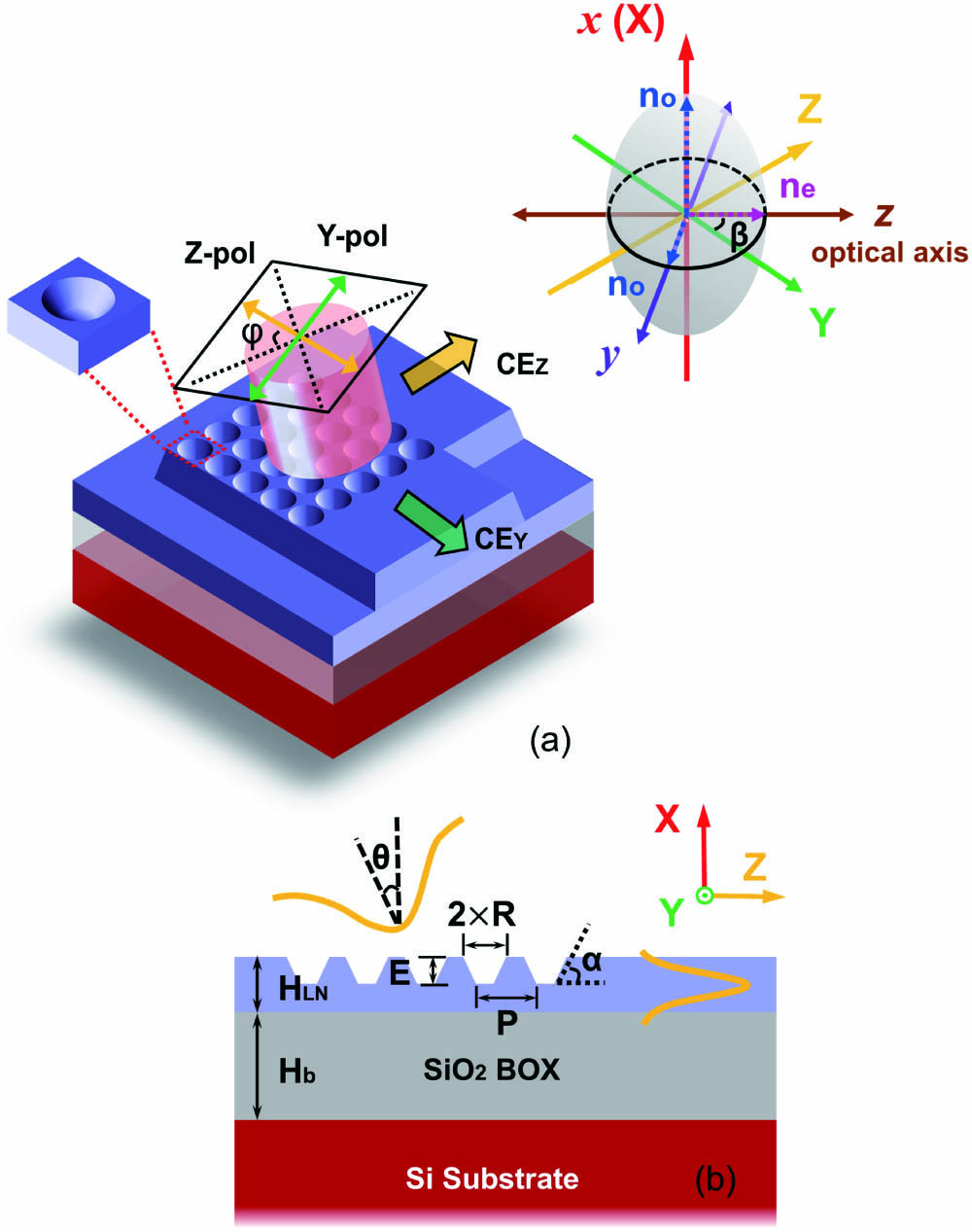
Set citation alerts for the article
Please enter your email address