
- Chinese Optics Letters
- Vol. 20, Issue 10, 100003 (2022)
Abstract
Keywords
1. Introduction
Biological imaging in the second near-infrared region (NIR-II, 1.0–1.7 µm) has been drawing considerable attention for many years due to its merits, including higher spatial resolution, deeper penetration depth, and lower optical absorption and scattering from biological tissues compared with those in the first near-infrared region (NIR-I, 650–950 nm) and visible regions[1–6]. As for the biological tissues, many of them, performing phase-only objects, are difficult to be observed or imaged accurately because only the phases, rather than the intensities, change when the light waves pass through the objects. Accordingly, bright-field imaging usually has too small contrasts to display accurately the details by imaging equipment or human eyes. Conventional phase-contrast (PC) methods[7] transform the phases into the intensity variations of the illumination signals, thus realizing the observations of phase samples. Nevertheless, the conventional PC has some shortcomings, e.g., the inevitable halo, fading phenomenon, and the limitation of the phase change to be much less than
In recent years, some efforts have been made to combine PC imaging with some nonlinear optical processes, including second harmonic generation (SHG)[17], sum-frequency generation (SFG)[18,19], and non-collinear optical parametric amplification (OPA) in order to achieve the DEE, thereby getting high imaging contrast and sensitivity. Especially for OPA, with the development of ultrafast laser technology, the shorter available pulse duration allows us to have higher OPA pump intensity, thereby achieving high gain and wide spatial bandwidth, which makes OPA imaging available for weaker illumination or imaging sensitivity, as well as higher spatial resolution[20,21]. In this paper, we use an intense 532 nm picosecond pulse as the OPA pump for high-spatial-resolution biological tissue imaging in the NIR-II region based on non-collinear OPA. In order to achieve the DEE, the pump has been modulated into a vortex laser pulse, while an OPA crystal is placed on the Fourier plane of a
2. Theory and Simulation
In principle, our imaging system is illustrated by Fig. 1, where a
Sign up for Chinese Optics Letters TOC. Get the latest issue of Chinese Optics Letters delivered right to you!Sign up now
Figure 1.Schematic of the spiral PC idler imaging system.
Here, the subscripts “
The symbol “
Figure 2 presents some numerical simulations for our spiral PC imaging system, as shown by Fig. 1 according to the subsequent experimental conditions. The pump is a 30 ps, 532 nm pulse with a pump intensity of
Figure 2.Theoretical simulations: (a) the spiral PC idler image of a phase-only object, (b) the traditional spiral PC image (without OPA), the idler images (c) with a Gaussian laser pump and (d) with a vortex laser pump of a quasi-phase object.
In Fig. 2, we aim to simulate the spiral PC idler imaging with the design of Fig. 1, which is illuminated by a weak 1064 nm signal. The used TA is a phase-only or a quasi-phase object with a spatial profile as a USAF 1951 resolution testing pattern. The quasi-phase object means it adds a week amplitude-modulation to its incident light beam. For a phase-only object, our simulation shows the OPA imaging pumped with traditional Gaussian laser is unable to capture TA information. If the pump is replaced by a vortex pulse (
3. Experimental Setup
Figure 3 shows the setup of the spiral PC idler imaging based on non-collinear OPA. The used light source is a commercial 10 Hz, 30 ps mode-locked neodymium-doped yttrium aluminum garnet (Nd:YAG) laser, which can simultaneously output two fundamental-mode Gaussian (
Figure 3.Setup of the spiral PC idler imaging. VOA, variable optical attenuator; M, mirror; TA, target; MS, microscope; L1–L5, optical lenses; DM, dichroic mirror; TDL, time delay line; SPP, spiral phase plate; NC, OPA crystal; CCD, CCD camera.
4. Experimental Results and Discussions
Firstly, a USAF 1951 pattern is used as a TA to calibrate the spatial resolution of our setup at absence of the MS and SPP. It is well known that the TA has many groups of spatial structures. Each group includes three parallel horizontal lines and three parallel vertical lines but corresponds to the same density. Figure 4(a1) is the idler image recorded via Gaussian-pumped OPA. It can display perfectly the three horizontal lines of Group 4.4 with a spatial feature of 22.62 lp/mm (44.2 µm), but only presents unclearly the three vertical lines. These phenomena can be well explained by Fig. 4(c1), where the one-dimensional (1D) intensity profile clearly shows three peaks across the three horizontal lines, but more than five (
Figure 4.Idler images: (a1) and (b1) with Gaussian pump, (a2) and (b2) with vortex pump, and (c1) and (c2) for the local 1D intensity profile of Group 4.4 marked with the white arrow in (a1) and (a2), respectively. Meanwhile, (a1) and (a2) for an intensity object (a USAF 1951 resolution testing pattern) and (b1) and (b2) for a phase object (a pattern formed by a piece of flat glass coated with UV curing adhesive).
To check the ability of our spiral PC idler imaging system for microscopic imaging, an MS is used behind the TA to distinguish more elaborate structures with an estimated axial resolution at 2 µm. We still calibrate the spatial resolution with the USAF 1951 pattern illuminated by a 1064 nm laser. Pumped with a Gaussian laser pulse (without SPF), from Fig. 5(a), we can see the parallel lines of Group 7.6 are well separated in the idler image, which is the highest density of our USAF 1951 pattern (228 lp/mm). If pumped with a vortex laser pulse, Fig. 5(b) can present the parallel lines more clearly with their bright and thin edges than Fig. 5(a) due to SPF or DEE. According to Fig. 5(b), it can be estimated that the idler PC image is able to resolve the TA details well beyond 228 lp/mm or 4.4 µm.
Figure 5.Idler images of the USAF 1951 resolution testing pattern with a 40× MS: (a) Gaussian laser pump and (b) vortex laser pump.
Figure 6 shows our spiral PC idler imaging for some biological tissues with their thickness at about 0.1 mm. The tissues are sandwiched between a pair of fixed transparent glass plates with the dimensions of
Figure 6.Microscopic images of frog egg cells and onion epidermis by (a1) and (b1) conventional MS imaging, (a2) and (b2) bright-field idler imaging, and (a3) and (b3) spiral PC idler imaging.
The used second sample is made of the onion epidermis. In order to avoid the disturbance from the multi-reflections, before the subsequent optical imaging, we record a microscopic image without anything at the imaged zone for calibration. Because the sizes of the glass plates are much larger than those of the biological tissues, the major zone of the gap between the fixed plate pair is filled with air, rather than the tissues. Each part of the plate pair is mounted on a translation stage when it is used. As a result, we can realize the calibration by aligning the translation stage so that the imaged zone is the air gap of the plate pair. The calibration finishes until the recorded optical field is so uniform that it is hard to see any fine structures, as shown in the right upper section (CI) of Fig. 6(b1). After this calibration, we move the translation stage so that the sample enters the imaged zone. Comparing this image with the calibration, we can distinguish the true TA structures from the artifacts. Similar to Figs. 6(a1)–6(a3), we have recorded three images with the
From the experimental results and discussions above, we can conclude that our system can present better microscopic imaging than both conventional idler imaging and MS imaging. We attribute the advantage to its high OPA gain and SPF. The latter provides us with both DEE and dark background.
5. Conclusions
In short, we have presented spiral PC idler imaging for biological tissues in the NIR-II region. Our setup includes a
References
[1] G. T. Kennedy, F. S. Azari, E. Bernstein, B. Nadeem, A. Chang, A. Segil, S. Carlin, N. T. Sullivan, E. Encarnado, C. Desphande, S. Kularatne, P. Gagare, M. Thomas, J. C. Kucharczuk, G. Christien, F. Lacombe, K. Leonard, P. S. Low, A. Criton, S. Singhal. Targeted detection of cancer at the cellular level during biopsy by near-infrared confocal laser endomicroscopy. Nat. Commun., 13, 2711(2022).
[2] Z. Feng, T. Tang, T. Wu, X. Yu, Y. Zhang, M. Wang, J. Zheng, Y. Ying, S. Chen, J. Zhou, X. Fan, D. Zhang, S. Li, M. Zhang, J. Qian. Perfecting and extending the near-infrared imaging window. Light Sci. Appl., 10, 197(2021).
[3] Y. Shi, Z. Zhang. Nonlinear photoacoustic imaging dedicated to thermal-nonlinearity characterization. Chin. Opt. Lett., 19, 071702(2021).
[4] K. Y. Zhang, Q. Yu, H. Wei, S. Liu, Q. Zhao, W. Huang. Long-lived emissive probes for time-resolved photoluminescence bioimaging and biosensing. Chem. Rev., 118, 1770(2018).
[5] R. Atchudan, T. N. J. I. Edisona, K. R. Aseer, S. Perumal, N. Karthik, Y. R. Lee. Highly fluorescent nitrogen-doped carbon dots derived from Phyllanthus acidus utilized as a fluorescent probe for label-free selective detection of Fe3+ ions, live cell imaging and fluorescent ink. Biosens. Bioelectron., 99, 303(2018).
[6] H. Zhou, X. Zeng, A. Li, W. Zhou, L. Tang, W. Hu, Q. Fan, X. Meng, H. Deng, L. Duan, Y. Li, Z. Deng, X. Hong, Y. Xiao. Upconversion NIR-II fluorophores for mitochondria-targeted cancer imaging and photothermal therapy. Nat. Commun., 11, 6183(2020).
[7] F. Zernike. Phase contrast, a new method for the microscopic observation of transparent objects. Physica, 9, 686(1942).
[8] J. Chen. Differential interference contrast. Opt. Instrum., 6, 1(1984).
[9] A. Edstrom, A. Lubk, J. Rusz. Quantum mechanical treatment of atomic-resolution differential phase contrast imaging of magnetic materials. Phys. Rev. B., 99, 174428(2019).
[10] P. Huo, C. Zhang, W. Zhu, M. Liu, S. Zhang, S. Zhang, L. Chen, H. J. Lezec, A. Agrawal, Y. Lu, T. Xu. Photonic spin-multiplexing metasurface for switchable spiral phase contrast imaging. Nano Lett., 20, 2791(2020).
[11] L. Li, Y. Zheng, H. Liu, X. Chen. Reconstitution of optical orbital angular momentum through strongly scattering media via feedback-based wavefront shaping method. Chin. Opt. Lett., 19, 100101(2021).
[12] J. Wang, J. Y. Yang, I. M. Fazal, N. Ahmed, Y. Yan, H. Huang, Y. Ren, Y. Yue, S. Dolinar, M. Tur, A. E. Willner. Terabit free-space data transmission employing orbital angular momentum multiplexing. Nat. Photonics, 6, 488(2012).
[13] T. Vicar, J. Balvan, J. Jaros, F. Jug, R. Kolar, M. Masarik, J. Gumulec. Cell segmentation methods for label-free contrast microscopy: review and comprehensive comparison. BMC Bioinform., 20, 360(2019).
[14] J. A. Davis, D. E. McNamara, D. M. Cottrell, J. Campos. Image processing with the radial Hilbert transform: theory and experiments. Opt. Lett., 25, 99(2000).
[15] M. Trusiak, M. Cywińska, V. Micó, J. Á. Picazo-Bueno, C. Zuo, P. Zdańkowski, K. Patorski. Variational Hilbert quantitative phase imaging. Sci. Rep., 10, 13955(2020).
[16] A. Jesacher, S. Furhapter, S. Bernet, M. Ritsch-Marte. Shadow effects in spiral phase contrast microscopy. Phys. Rev. Lett., 94, 233902(2005).
[17] X. Qiu, F. Li, W. Zhang, Z. Zhu, L. Chen. Spiral phase contrast imaging in nonlinear optics: seeing phase objects using invisible illumination. Optica, 5, 208(2018).
[18] S. K. Liu, C. Yang, S. L. Liu, Z. Y. Zhou, Y. Li, Y. H. Li, Z. H. Xu, G. C. Guo, B. S. Shi. Up-conversion imaging processing with field-of-view and edge enhancement. Phys. Rev. Appl., 11, 044013(2019).
[19] S. Junaid, P. Tidemand-Lichtenberg, C. Pedersen, P. J. Rodrigo. Upconversion dark-field imaging with extended field of view at video frame rate. Appl. Opt., 59, 2157(2020).
[20] X. Zeng, Y. Cai, W. Chen, S. Zheng, J. Li, T. Zhu, S. Xu. High spatially resolved idler image with a compact non-collinear optical parametric amplifier using a CW laser as signal. IEEE Photonics J., 7, 6804107(2015).
[21] X. Zeng, Y. Cai, W. Chen, J. Li, S. Zheng, T. Zhu, S. Xu. High resolved non-collinear idler imaging via type-II angular noncritical phase-matching. IEEE Photon. Technol. Lett., 28, 2685(2016).
[22] I. V. Sokolov, M. I. Kolobov, L. A. Lugiato. Quantum fluctuations in traveling-wave amplification of optical images. Phys. Rev. A, 60, 2420(1999).
[23] C. S. Guo, Y. J. Han, J. B. Xu, J. Ding. Radial Hilbert transform with Laguerre-Gaussian spatial filters. Opt. Lett., 31, 1394(2006).
[24] S. Furhapter, A. Jesacher, S. Bernet, M. Ritsch-Marte. Spiral phase contrast imaging in microscopy. Opt. Express, 13, 689(2005).
[25] R. W. Boyd. Nonlinear Optics(2002).
[26] G. Agrawal. Nonlinear Fiber Optics(2013).
[27] P. A. Morris, R. S. Aspden, J. E. Bell, R. W. Boyd, M. J. Padgett. Imaging with a small number of photons. Nat. Commun., 6, 5913(2015).
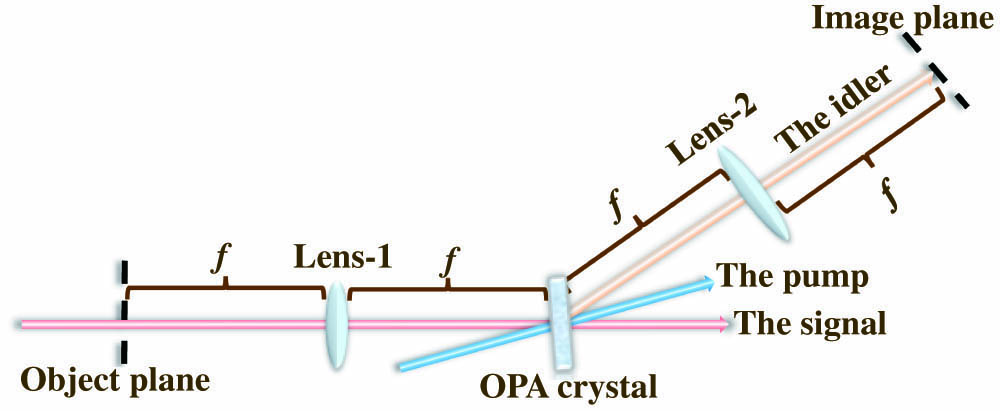
Set citation alerts for the article
Please enter your email address