Jian Liu, Ka-Di Zhu, "Room temperature optical mass sensor with an artificial molecular structure based on surface plasmon optomechanics," Photonics Res. 6, 867 (2018)

Search by keywords or author
- Photonics Research
- Vol. 6, Issue 9, 867 (2018)
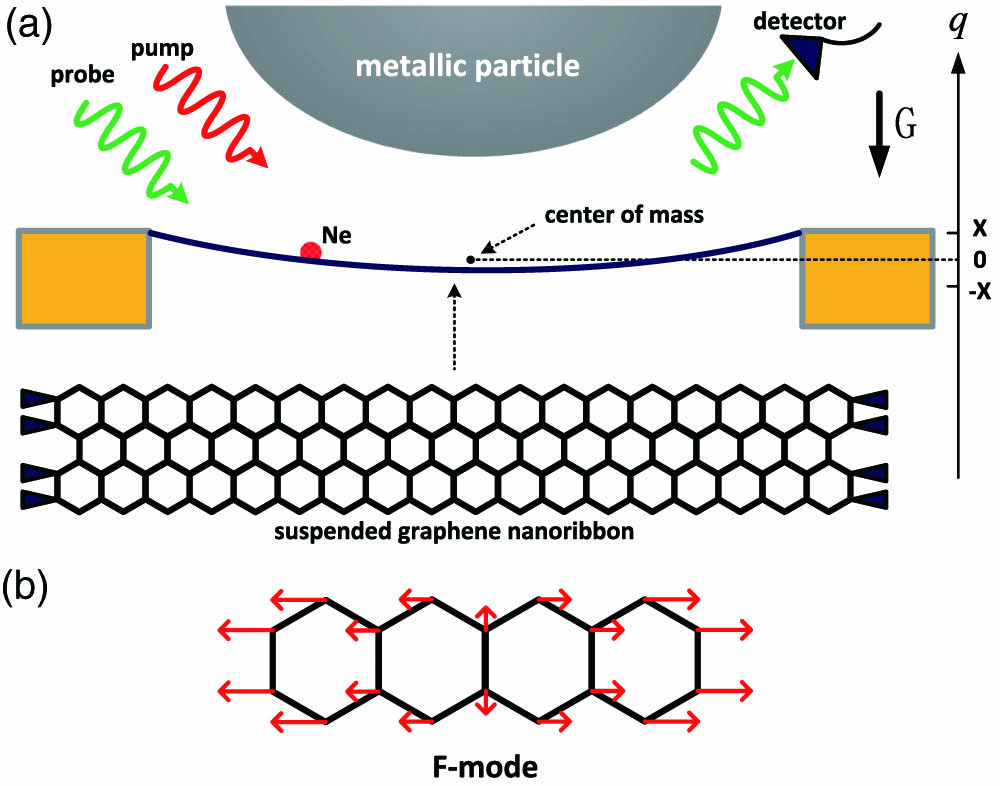
Fig. 1. (a) Schematic diagram of the suspended graphene nanoribbon placed in the surface plasmonic cavity with presence of a strong pump beam and a weak probe beam; G points the direction of the gravity. The Ne atoms are deposited onto the surface of the graphene sheet in a special evaporator. (b) Displacement pattern of the atoms in the graphene nanoribbon due to the fundamental in-plane flexural resonance mode.
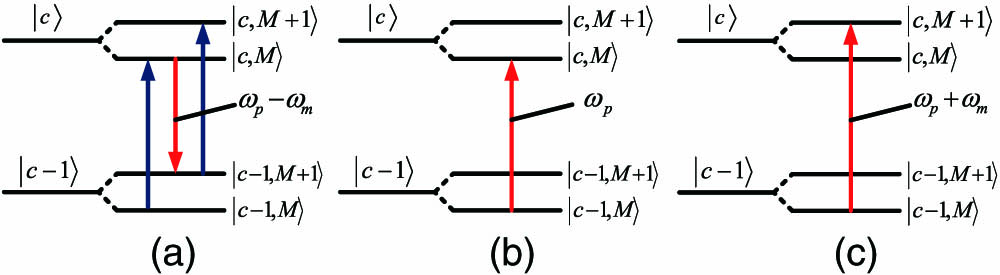
Fig. 2. Energy level diagram of the SGR-plasmon optomechanical system, where M and c denote the number states of mechanical mode and plasmon cavity photon, respectively. The three pictures correspond to the physical processes of (a) Stokes scattering, (b) Rayleigh scattering, and (c) anti-Stokes scattering.
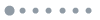
Fig. 3. Strength of Rayleigh scattering on the probing absorption spectrum as a function of the probe-pump detuning δ c for different quality factors of the plasmon. We set E p = 0 ; other parameter values are Ω s = 0.1 THz , γ = 0.5 GHz .
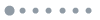
Fig. 4. Plot of absorption spectrum as a function of probe-pump detuning with R = 10 3 Å 4 · amu − 1 , g = 200 GHz , Q c = 10 , and Δ p = 0 for I = 1 , 2 , and 3 kW / cm 2 , respectively. Other parameter values are the same as in Fig. 3 .
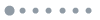
Fig. 5. Pump intensity dependence of the ratio between Raman and Rayleigh scattering strength with different optomechanical coupling rate g .
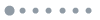
Fig. 6. Absorption spectra of the probe pulse as a function of δ before (black line) and after the binding events of one Ne atom (blue line) and 10 atoms (red line). The frequency shifts induced by additional masses can be well distinguished in the spectra. Here we choose R = 10 3 Å 4 · amu − 1 , I = 1 kW / cm 2 . Other parameters used are the same as in Fig. 4 .
|
Table 1. Parameters of the Plasmon Optomechanical System Used in the Mass Measurement
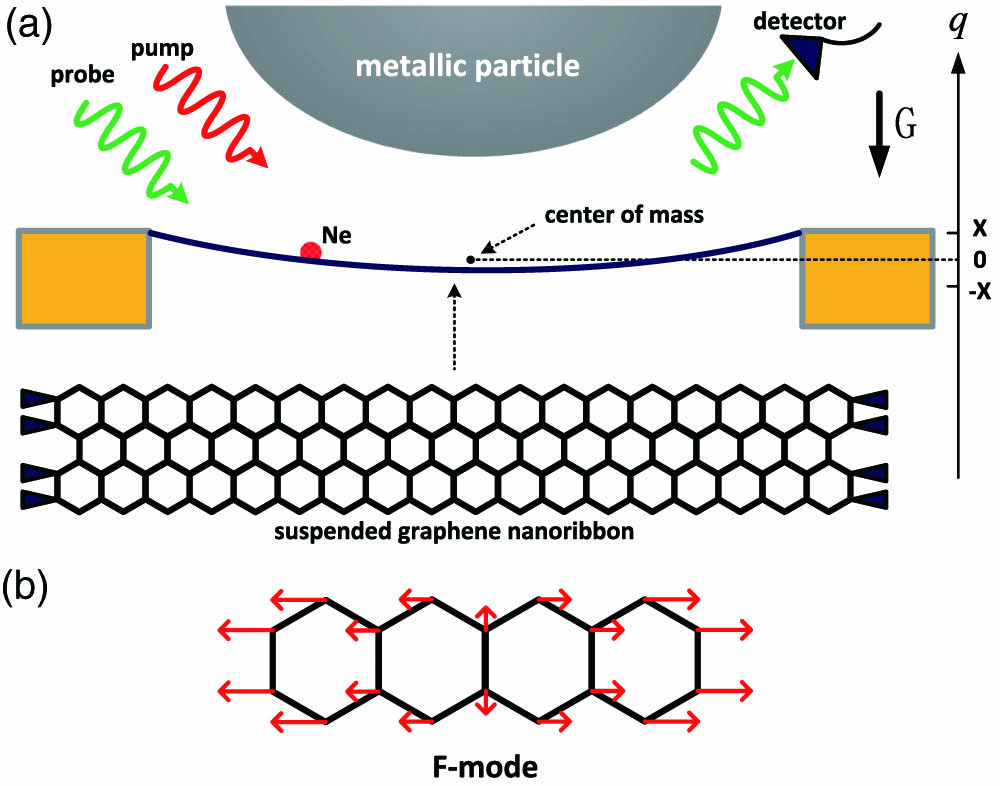
Set citation alerts for the article
Please enter your email address