
- Photonics Research
- Vol. 10, Issue 2, 297 (2022)
Abstract
1. INTRODUCTION
Recently, optical sorting based on an optical waveguide has demonstrated tremendous potential for applications in pharmacies [1], diagnosis of cancer cells [2,3], purification of water [4], etc., due to the advantages of scalability, long-range manipulation, high throughput, high capacity, and easy integration with lab-on-a-chip systems [2,5–9]. In these manipulations, an optical force can sustainedly propel a variety of microparticles such as dielectric microparticles [10], metallic nanoparticles [11,12], and cells [13], and sort these microparticles [14].
In order to make the optical sorting more efficient, it is better to make the object resonant with the light source [15–17]. It is reported that the optical force on microparticles under resonant conditions can approach nearly the limit of totally absorbing particles [15]. And the propulsion velocities of the microparticles can accelerate 1 order faster than the previous observations in nonresonance [5,13,18–21]. Unfortunately, however, the resonant optical sorting with enhanced optical forces is not suitable for multiparticle optical sorting in waveguide structures. For the widely used optical sorting using a dielectric optical waveguide, as shown in Fig. 1(a), the second particle cannot be manipulated efficiently anymore (i.e.,
Figure 1.Schematic and performances of resonant optical manipulation using the traditional optical waveguide. (a) Schematic of optical manipulation based on a strip waveguide (GaAs). The parameters are
In order to improve the transmittance and reduce the mode disturbance, the edge-to-edge distance
Sign up for Photonics Research TOC. Get the latest issue of Photonics Research delivered right to you!Sign up now
In order to overcome this drawback, in this paper, we propose to perform optical sorting using a topological photonic crystal (PC) structure, which supports a topology-protected guiding mode [25,26], as shown in Fig. 2(a). Because of the topology-protected feature, the propagation of the light field is immune to the local disorders, including the objects to be sorted [Fig. 2(b)]. Thus, the optical sorting mechanism proposed here is extremely efficient and has a high throughput for multiparticle optical sorting.
Figure 2.Schematic of resonant optical manipulation using a topology-protected guiding channel. (a) The proposed new system is based on a topological photonic structure (the gray substrate), which supports a topology-protected guiding mode. Both sphere 1 and sphere 2 (the red spheres) are resonantely repelled from the substrate. (b) When particle 1 resonantly interacts with the incident light, a large repulsive force
2. RESULTS AND DISCUSSION
A. Topological Photonic Slabs
As an illustration of example, here we use a honeycomb photonic crystal to achieve the topological photonic structures by deforming the honeycomb lattice [27]. Figure 3(a) shows the schematic of the topological photonic crystal structure, which is implemented on a GaAs dielectric substrate. We start from a honeycomb lattice with the hexagonal clusters composed of six neighboring equilateral triangular holes (white) with a side length of
Figure 3.Structure, parameter, and performance of the topologically protected guiding channel. (a) Schematic of the topological photonic structure composed of two different honeycomb lattices.
Figure 3(b) shows the band structure of the TE modes along the
Using the three-dimensional finite difference time domain (FDTD) method, we get the propagation properties of light in the trapezoidal structure composed of expanded and shrunken structures, as shown in Fig. 3(c). The structure is excited by a circularly polarized electric dipole (at the frequency of 315 THz) located at the position marked in Fig. 3(a). The light propagation is strictly confined to the interface and can be perfectly transmitted without obvious loss or backscattering even through four cascade sharp turns. It is noted that the topological edge state is broadband and can maintain robust transmission from 300 to 323 THz.
B. Optical Force of Particles on the Topological Photonic Slabs
Now, let us quantitatively analyze the optical force on the particles when they are near the topological PC structure, as illustrated in Fig. 2(a). Without loss of generality, the particles are made of
The resonance condition is satisfied for a series of radii, which is known as morphology dependent resonances (MDRs) [30]. The MDR is related to the incident light frequency, and the resonance shifts when the light frequency changes. Since the optical force peak repeats periodically with the particle radius, only the values of
Figure 4.Optical forces on two spheres using the topological structure designed in Fig.
At the resonant point A marked in Fig. 4(a1),
Figure 4(b) shows the electric field distributions along the
For the optical sorting of multiple particles, the distance
In order to compare the multiparticle manipulation efficiencies of the topological and ordinary waveguide structures, 10 identical particles are introduced for illustration, and their ratios of
C. Multiparticle Optical Sorting
From Fig. 4(a2), one can find that the sign of
In a liquid environment, it should be noted that the particles are subjected to several other forces in addition to the optical force in the process of movement, including Brownian force, drag force, gravity, and buoyancy. For simplicity, the particles are placed in a static water environment, the drag force due to the flow of the liquid and the resistance provided by the shear flow are ignored [31,32], and only the drag force caused by the particles’ movement is considered. In addition, the contributions of gravity (
In order to analyze the feasibility of multiparticle resonant optical sorting in the topological photonic structure, three types of particles [corresponding to A, B, C marked in Fig. 4(a)] were mixed and placed randomly on the surface of the slab, as shown in Fig. 5(a). To understand the optical sorting along the
Figure 5.Dynamics of the resonant multiparticle sorting using the topological photonic slab. (a) Schematic of multiparticle optical sorting scheme using the topological structure, where a mixture of the three types of particles A, B, C marked in Fig.
The time evolution dynamics of the particles on the topological photonic slab shown in Fig. 5(a) is numerically analyzed using the Eqs. (2)–(4). For the particles of type A (the red spheres), they experience repulsive forces in the
Taking the particle
From the trajectories of particles, it can be seen that the particles with the radius
The proposed optical sorting scheme could be implemented in a topological PC structure, which can be fabricated by electron beam lithography [39]. By introducing quantum dots or a standard waveguide, the topological edge states with robustness and broad bandwidth operation can be guaranteed [40–42]. In particular, the optical guiding of submicrometer dielectric particles on the photonic crystal waveguides has been achieved experimentally in Ref. [43]. In short, the feasibility of optical sorting using a topological photonic structure and its application in the biological field are still open, which should motivate further research on this topic [44]. Further, combining it with microfluidic chips to build miniaturized and portable biochemical detection devices is also a possible direction of future research [45].
3. CONCLUSION
In conclusion, we have proposed a powerful optical sorting platform using a topological photonic structure, which supports a topology-protected optical mode immune to local disorders and the particles to be manipulated. Based on this merit, an efficient optical sorting scheme for multiple and parallel sorting is proposed and investigated numerically. This scheme offers several advantages. First, this sorting method is realized at resonance, which utilizes the laser power to enhance the optical force and improve the sorting efficiency. Second, it supports high throughput and parallel sorting manipulation, as the topological protection property ensures that a large number of resonant particles can be manipulated simultaneously. Last but not the least, the topological slab is well suited for integration with a lab-on-a-chip platform. Although only a pilot demonstration of this new optical sorting mechanism is performed, it paves the way for future experimental study of efficient optical sorting based on chip integration for biophysical and biochemical analysis.
References
[1] J. M. Hou, M. G. Krebs, L. Lancashire, R. Sloane, A. Backen, R. K. Swain, L. J. C. Priest, A. Greystoke, C. Zhou, K. Morris, T. Ward, F. H. Blackhall, C. Dive. Clinical significance and molecular characteristics of circulating tumor cells and circulating tumor microemboli in patients with small-cell lung cancer. J. Clin. Oncol., 30, 525-532(2012).
[2] M. M. Wang, E. Tu, D. E. Raymond, J. M. Yang, H. C. Zhang, N. Hagen, B. Dees, E. M. Mercer, A. H. Forster, I. Kariv, P. J. Marchand, W. F. Butler. Microfluidic sorting of mammalian cells by optical force switching. Nat. Biotechnol., 23, 83-87(2004).
[3] N. M. Karabacak, P. S. Spuhler, F. Fachin, E. J. Lim, V. Pai, E. Ozkumur, J. M. Martel, N. Kojic, K. Smith, P. Chen, J. Yang, H. Hwang, B. Morgan, J. Trautwein, T. A. Barber, S. L. Stott, S. Maheswaran, R. Kapur, D. A. Haber, M. Toner. Microfluidic, marker-free isolation of circulating tumor cells from blood samples. Nat. Protoc., 9, 694-710(2014).
[4] A. Atajanov, A. Zhbanov, S. Yang. Sorting and manipulation of biological cells and the prospects for using optical forces. Micro Nano Syst. Lett., 6, 2(2018).
[5] A. H. J. Yang, S. D. Moore, B. S. Schmidt, M. Klug, M. Lipson, D. Erickson. Optical manipulation of nanoparticles and biomolecules in sub-wavelength slot waveguides. Nature, 457, 71-75(2009).
[6] R. W. Applegate, J. Squier, T. Vestad, J. Oakey, D. W. M. Marr, P. Bado, M. A. Dugand, A. A. Said. Microfluidic sorting system based on optical waveguide integration and diode laser bar trapping. Lab Chip, 6, 422-426(2006).
[7] H. Li, Y. Y. Cao, B. J. Shi, T. T. Zhu, Y. Geng, R. Feng, L. Wang, F. K. Sun, Y. Z. Shi, M. A. Miri, M. N. Vesperinas, C. W. Qiu, W. Q. Ding. Momentum-topology-induced optical pulling force. Phys. Rev. Lett., 124, 143901(2020).
[8] H. Li, Y. Y. Cao, L. M. Zhou, X. H. Xu, T. T. Zhu, Y. Z. Shi, C. W. Qiu, W. Q. Ding. Optical pulling forces and their applications. Adv. Opt. Photon., 12, 288-366(2020).
[9] T. T. Zhu, Y. Y. Cao, L. Wang, Z. Q. Nie, T. Cao, F. K. Sun, Z. H. Jiang, C. W. Qiu, W. Q. Ding. Self-induced backaction optical pulling force. Phys. Rev. Lett., 120, 123901(2018).
[10] L. N. Ng, B. J. Luf, M. N. Zervas, J. S. Wilkinson. Forces on a Rayleigh particle in the cover region of a planar waveguide. J. Lightwave Technol., 18, 388-400(2000).
[11] S. Gaugiran, S. Gétin, J. M. Fedeli, J. Derouard. Polarization and particle size dependence of radiative forces on small metallic particles in evanescent optical fields. Evidences for either repulsive or attractive gradient forces. Opt. Express, 15, 8146-8156(2007).
[12] L. N. Ng, B. J. Luff, M. N. Zervas, J. S. Wilkinson. Propulsion of gold nanoparticles on optical waveguides. Opt. Commun., 208, 117-124(2002).
[13] S. Gaugiran, S. Gétin, J. M. Fedeli, G. Colas, A. Fuchs, F. Chatelain, J. Dérouard. Optical manipulation of microparticles and cells on silicon nitride waveguides. Opt. Express, 13, 6956-6963(2005).
[14] Y. Z. Shi, S. Xiong, L. K. Chin, J. B. Zhang, W. Ser, J. H. Wu, T. N. Chen, Z. C. Yang, Y. L. Hao, B. Liedberg, P. H. Yap, D. P. Tsai, C. W. Qiu, A. Q. Liu. Nanometer-precision linear sorting with synchronized optofluidic dual barriers. Sci. Adv., 4, eaao0773(2018).
[15] Y. C. Li, O. V. Svitelskiy, A. V. Maslov, D. Carnegie, E. Rafailov, V. N. Astratov. Giant resonant light forces in microspherical photonics. Light Sci. Appl., 2, e64(2013).
[16] S. Y. Lin, E. Schonbrun, K. Crozier. Optical manipulation with planar silicon microring resonators. Nano Lett., 10, 2408-2411(2010).
[17] A. Einat, U. Levy. Analysis of the optical force in the micro ring resonator. Opt. Express, 19, 20405-20419(2011).
[18] K. Grujica, O. G. Hellesø, J. S. Wilkinson, J. P. Hole. Optical propulsion of microspheres along a channel waveguide produced by Cs+ ion-exchange in glass. Opt. Commun., 239, 227-235(2002).
[19] B. S. Schmidt, A. H. J. Yang, D. Erickson, M. Lipson. Optofluidic trapping and transport on solid core waveguides within a microfluidic device. Opt. Express, 15, 14322-14334(2007).
[20] G. Brambilla, G. S. Murugan, J. S. Wilkinson, D. J. Richardson. Optical manipulation of microspheres along a subwavelength optical wire. Opt. Lett., 32, 3041-3043(2007).
[21] R. F. Marchington, M. Mazilu, S. Kuriakose, V. Garcés-Chávez, P. J. Reece, T. F. Krauss, M. Gu, K. Dholakia. Optical deflection and sorting of microparticles in a near-field optical geometry. Opt. Express, 16, 3712-3726(2008).
[22] G. S. Wiederhecker, L. Chen, A. Gondarenko, M. Lipson. Controlling photonic structures using optical forces. Nature, 462, 633-636(2009).
[23] M. L. Povinelli, M. Loncar, M. I. Banescu, E. J. Smythe, J. D. Joannopoulos. Evanescent-wave bonding between optical waveguides. Opt. Lett., 30, 3042-3044(2005).
[24] P. T. Rakich, M. A. Popović, M. Soljačić, E. P. Ippen. Trapping, corralling and spectral bonding of optical resonances through optically induced potentials. Nat. Photonics, 1, 658-665(2007).
[25] L. Lu, J. D. Joannopoulos, M. Soljačić. Topological photonics. Nat. Photonics, 8, 821-829(2014).
[26] A. B. Khanikaev, G. Shvets. Two-dimensional topological photonics. Nat. Photonics, 11, 763-773(2017).
[27] S. Barik, H. Miyake, W. DeGottardi, E. Waks, M. Hafezi. Two-dimensionally confined topological edge states in photonic crystals. New J. Phys., 18, 113013(2016).
[28] L. H. Wu, X. Hu. Scheme for achieving a topological photonic crystal by using dielectric material. Phys. Rev. Lett., 114, 223901(2015).
[29] X. D. Chen, X. T. He, J. W. Dong. All-dielectric layered photonic topological insulators. Laser Photon. Rev., 13, 1900091(2019).
[30] H. Y. Jaising, O. G. Hellesø. Radiation forces on a Mie particle in the evanescent field of an optical waveguide. Opt. Commun., 246, 373-383(2005).
[31] G. P. Krishnan, D. T. Leighton. Inertial lift on a moving sphere in contact with a plane wall in a shear flow. Phys. Fluids, 7, 2538-2545(1995).
[32] A. J. Goldman, R. G. Cox, H. Brenner. Slow viscous motion of a sphere parallel to a plane wall—II Couette flow. Chem. Eng. Sci., 22, 653-660(1967).
[33] D. A. Shilkin, E. V. Lyubin, M. R. Shcherbakov, M. Lapine, A. A. Fedyanin. Directional optical sorting of silicon nanoparticles. ACS Photon., 4, 2312-2319(2017).
[34] W. H. Xu, Y. Y. Wang, W. X. Jiao, F. Wang, X. F. Xu, M. Jiang, H. P. Ho, G. H. Wang. Tunable optofluidic sorting and manipulation on micro-ring resonators from a statistics perspective. Opt. Lett., 44, 3226-3229(2019).
[35] L. Wang, Y. Y. Cao, B. J. Shi, H. Li, R. Feng, F. K. Sun, L. Y. Lin, W. Q. Ding. Subwavelength optical trapping and transporting using Bloch mode. Opt. Lett., 45, 1886-1889(2020).
[36] Y. Z. Shi, S. Xiong, Y. Zhang, L. K. Chin, Y. Y. Chen, J. B. Zhang, T. H. Zhang, W. Ser, A. Larrson, S. H. Lim, J. H. Wu, T. N. Chen, Z. C. Yang, Y. L. Hao, B. Liedberg, P. H. Yap, K. Wang, D. P. Tsai, C. W. Qiu, A. Q. Liu. Sculpting nanoparticle dynamics for single-bacteria-level screening and direct binding-efficiency measurement. Nat. Commun., 9, 815(2018).
[37] E. E. Michaelides. Brownian movement and thermophoresis of nanoparticles in liquids. Int. J. Heat Mass Transfer, 81, 179-187(2015).
[38] Y. Zhang, B. J. Li. Particle sorting using a subwavelength optical fiber. Laser Photon. Rev., 7, 289-296(2013).
[39] C. P. Reardon, I. H. Rey, K. Welna, L. O’Faolain, T. F. Krauss. Fabrication and characterization of photonic crystal slow light waveguides and cavities. J.Vis. Exp., 69, e50216(2012).
[40] S. Barik, A. Karasahin, C. Flower, T. Cai, H. Miyake, W. DeGottardi, M. Hafezi, E. Waks. A topological quantum optics interface. Science, 359, 666-668(2018).
[41] X. T. He, E. T. Liang, J. J. Yuan, H. Y. Qiu, X. D. Chen, F. L. Zhao, J. W. Dong. A silicon-on-insulator slab for topological valley transport. Nat. Commun., 10, 872(2019).
[42] M. I. Shalaev, W. Walasik, A. Tsukernik, Y. Xu, N. M. Litchinitser. Robust topologically protected transport in photonic crystals at telecommunication wavelengths. Nat. Nanotechnol., 14, 31-34(2019).
[43] M. G. Scullion, Y. Arita, T. F. Krauss, K. Dholakia. Enhancement of optical forces using slow light in a photonic crystal waveguide. Optica, 2, 816-821(2015).
[44] M. Jung, R. G. Gladstone, G. Shvets. Nanopolaritonic second-order topological insulator based on graphene plasmons. Adv. Photon., 2, 046003(2020).
[45] Y. J. Yang, Y. X. Ren, M. Z. Chen, Y. Arita, C. R. Guzmán. Optical trapping with structured light: a review. Adv. Photon., 3, 034001(2021).
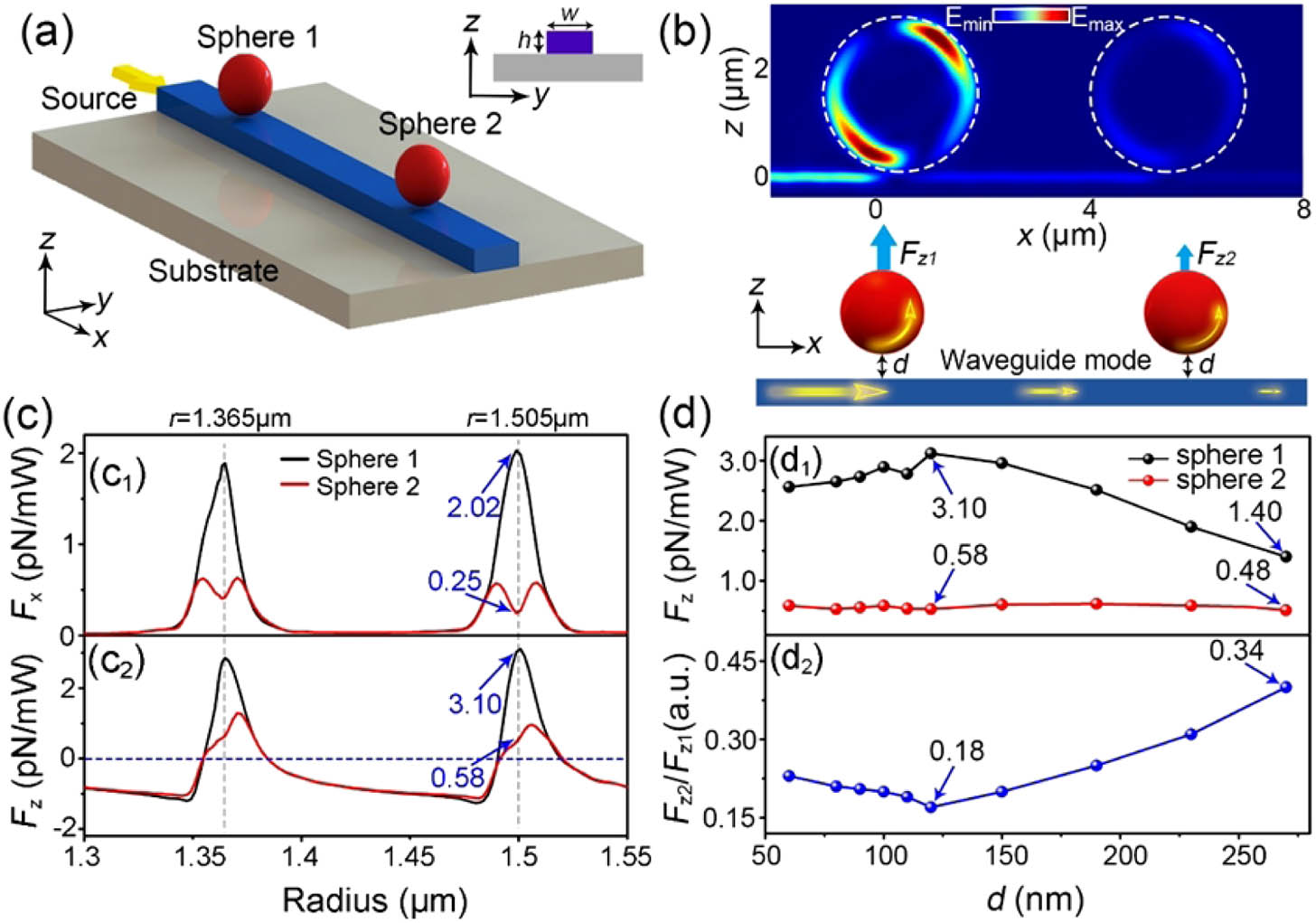
Set citation alerts for the article
Please enter your email address