
- Chinese Optics Letters
- Vol. 19, Issue 12, 123201 (2021)
Abstract
Keywords
1. Introduction
The generation of the ultrashort optical pulses in the extreme ultraviolet (XUV) range has allowed the electron’s ultrafast dynamic processes in atoms[
An as XUV pulse train or a single XUV pulse is widely applied to investigate as time delays of photoemission when they are combined with a phase-locked IR field. When an as train is combined with an IR field, this scheme is often referred to as the reconstruction of as beating by interference of two-photon transition (RABBITT)[
It is known that the photoionization processes in the as streaking technique and the RABBITT technique are laser-assisted, where an electron is ionized in the superposition of the XUV and IR fields. The effect of the IR field on the electron dynamics cannot be neglected[
Sign up for Chinese Optics Letters TOC. Get the latest issue of Chinese Optics Letters delivered right to you!Sign up now
In this paper, we mainly study the effects of the IR field on the dynamics of the electron emitted by a double-XUV pulse alone and in the presence of an IR field using a Wigner distribution-like (WDL) function based on the strong field approximation (SFA) theory.
2. Theory Method
The first term of the
Here,
Substituting Eq. (2) into Eq. (1), we can get
The detailed definition and derivation of the WDL function can be found in Refs. [29,30]. Here, we directly give the WDL function in one-dimensional system, which is written as
The WDL function satisfies the following relationship:
The energy spectrum of photoelectrons can be given exactly by Eq. (6) in the one-dimensional system.
Further, the ionization time distribution (ITD)
In this paper, the vector potential of two XUV pulses in the presence of an IR field is given by
Here,
Here, the subscripts
In the present work, we mainly adopt a model atom with
3. Results and Discussions
In Fig. 1(a), we first calculate the time-energy distributions (TEDs) of electrons emitted along the positive
Figure 1.(a) TEDs of electrons along the positive x axis (Θ = 0) emitted by two XUV pulses (φXUV = 0) separated by a half-cycle of an 800 nm IR field. Panels (b) and (c) show the ITDs of electrons obtained by integrating the TED over the energy for φXUV = 0 and φXUV = 0.5π, respectively. The corresponding electric fields of the two XUV pulses are also given (gray dashed dotted line).
Figure 2.Frequency spectrum of the double-XUV pulse with parameters like those of Fig.
Furthermore, the ITDs of electrons along the positive (black solid line) and negative (red solid line) directions are given by integrating the TED over the energy in Fig. 1(b) for
The shape of the ITD mainly resembles the XUV pulse envelope, meanwhile showing the dependence on the CEP of the XUV pulse and the electron’s emission direction [see the insets in Figs. 1(b) and 1(c)]. We take the ITD during the first XUV pulse as an example to give a brief description (see Ref. [26] for more details). For
Next, we investigate the dynamics of electrons ionized by a double-XUV pulse (
Figure 3.Electric fields of two XUV pulses with φXUV = 0 (gray solid line) and an 800 nm IR (red solid line) pulse. (a) Case one: the center of the XUV pulse coincides with the zero crossings of the IR field amplitude. (b) Case two: the center of the XUV pulse coincides with the maximum of the IR field amplitude. The blue dashed lines represent the sum of the two XUV and IR fields, and the black dashed lines denote the negative vector potential of the IR field. Magenta (green) dotted lines denote the electric field of the XUV pulse that is enhanced (suppressed) after adding the IR field. Here, IIR = 1 × 1013 W/cm2.
Figure 4 shows the TEDs of photoelectrons emitted along the positive (left column) and negative (right column) directions in the two-color field of case one for three different IR intensities of
Figure 4.TEDs of electrons emitted along the positive (left column) and negative (right column) directions in the two-color field of case one. The intensities of the IR field are (a), (b) 1 × 1011 W/cm2, (c), (d) 1 × 1012 W/cm2, and (e), (f) 1 × 1013 W/cm2, respectively. The white solid line denotes the energy spectrum.
When the IR intensity is low (
In Fig. 5, similar calculations are performed for the two-color field of case two. The parameters are the same as in Fig. 4, except for
Figure 5.TEDs of electrons emitted along the positive (left column) and negative (right column) directions in the two-color field of case two. The intensities of the IR field are (a), (b) 1 × 1011 W/cm2, (c), (d) 1 × 1012 W/cm2), and (e), (f) 1 × 1013 W/cm2, respectively. The white solid lines denote the energy spectra.
In contrast to the TED shown in Fig. 4, another distinct difference is that with increasing IR intensity, the interval between the adjacent interference peaks gradually increases in the positive direction and decreases in the negative direction. This is attributed to the fact that the electrons generated during two XUV pulses have different accumulated phases, which depend on the vector potential
From Figs. 4 and 5, we can find that with increasing IR intensity the TED clearly and intuitively shows either the energy shift of the photoelectron or the change of the interval of the interference structure for the different two-color fields.
In Fig. 6, we integrate the TEDs shown in Figs. 4 and 5 over the energy to obtain the ITDs. Figures 6(a) and 6(b) [Figs. 6(c) and 6(d)] display the ITDs of the electron emitted in the positive and negative directions, respectively, for the two-color field of case one (case two). To see clearly, each peak of the ITD is enlarged in the inset of the corresponding panel. By comparing Fig. 1(b) with Fig. 6, the obvious feature is that the ITD during the first XUV pulse is not the same as that during the second one, and the ITD during each XUV pulse is no longer symmetric with respect to the central axis of the corresponding XUV pulse, regardless of the electron’s emission direction. The direct reason is that the introduction of the IR field causes the different changes of the electric field during the two XUV pulses (see Fig. 3), which will be discussed below.
Figure 6.ITDs of the photoelectron emitted along the positive (left column) and negative (right column) directions for the two-color fields of (a), (b) case one and (c), (d) case two. The peak of the ITD is enlarged in the inset.
For the two-color field of case one shown in Fig. 3(a), after adding the IR field, the central one-cycle field during the first XUV pulse is increased (see magenta dotted line), while the corresponding electric field during the second XUV pulse is decreased (see green dotted line). This leads to the enhanced (suppressed) ionization rate of electrons generated during the first (second) XUV pulse, as shown in Figs. 6(a) and 6(b). The stronger the IR intensity is, the higher (lower) the ionization rate for the first (second) XUV pulse is. Further, give a closer look at the peaks of the insets in Figs. 6(a) and 6(b). For each ITD, when the IR intensity is high (low), the shape of the ITD exhibits asymmetric (approximately symmetric) with respect to the center of the corresponding XUV pulse. This is because the relation of
For the two-color field of case two shown in Fig. 3(b), the IR field clearly causes the enhancement of the electric field on one side of each XUV pulse envelope, while the suppression of the electric field on the other side of the envelope, namely,
From Fig. 6, one can find that the introduction of the IR field changes the electron’s emission time, and the value of the change becomes larger and larger with increasing IR intensity. Moreover, it is found that the ITD during one XUV pulse is only related to the electric field of the corresponding XUV pulse in the presence of the IR field. In other words, it is uncorrelated with the other XUV pulse.
From a semiclassical point of view, when the value of
Figure 7.TEDs of the photoelectron generated from an atom with IP = 1.3 a.u. by two XUV pulses in the presence of a 1600 nm IR field with IIR = 1 × 1013 W/cm2 in the (a) positive and (b) negative directions. The two-color field is the same as case one shown in Fig.
4. Conclusion
In conclusion, we use a WDL function to calculate the TEDs and ITDs of photoelectrons emitted by a double-XUV pulse and a 2XUV-IR two-color pulse. In the case of a double-XUV pulse, besides two broad distributions during two XUV pulses, there are many horizontal fringes in the TED that come from the interference between electrons generated, respectively, by two XUV pulses with the same energy. Moreover, it can be found that the TED and ITD of the electron ionized by the first XUV pulse are the same as the corresponding distributions generated by the second XUV pulse, which are also the same as those in the case of a single XUV pulse.
In the case of a double-XUV pulse combined with an IR field, the effect of the IR field on the electron dynamics is clearly presented in the TED and ITD. The TED intuitively shows that the IR field can cause either the energy shift of the photoelectron or the change of energy interval of the interference fringes due to the different accumulated phase of electrons ionized during two XUV pulses. The change in the shape of the ITD can be explained by the change of the electric field. Further, our calculation shows the ITD of the electron ionized during each XUV pulse is equivalent to that in the case involving XUV pulses combined with an IR field, which is not affected by the other XUV pulse. We may infer that for an APT combined with an IR field, the effect of the IR field on the emission time of the electron ionized during each XUV pulse mainly depends on the electric field of the involved XUV field combined with the IR field.
Finally, we calculate the TED of photoelectrons emitted from an atom with higher ionization energy to further check the validity of the semiclassical theory in the description of the photoelectron emitted by a double-XUV pulse combined with a low or moderate IR field. In addition, the results calculated by the WDL function are in the time domain, which are hard to directly measure in the current experiments. We believe that it is possible to measure them in future experiments with higher temporal measuring accuracy.
References
[1] M. Drescher, M. Hentschel, R. Kienberger, M. Uiberacker, V. Yakovlev, A. Scrinzi, Th. Westerwalbesloh, U. Kleineberg, U. Heinzmann, F. Krausz. Time-resolved atomic inner-shell spectroscopy. Nature, 419, 803(2002).
[2] K. Klünder, J. M. Dahlström, M. Gisselbrecht, T. Fordell, M. Swoboda, D. Guénot, P. Johnsson, J. Caillat, J. Mauritsson, A. Maquet, R. Taïeb, A. L’Huillier. Probing single-photon ionization on the attosecond time scale. Phys. Rev. Lett., 106, 143002(2011).
[3] C. Ott, A. Kaldun, L. Argenti, P. Raith, K. Meyer, M. Laux, Y. Zhang, A. Blättermann, S. Hagstotz, T. Ding, R. Heck, J. Madroñero, F. Martín, T. Pfeifer. Reconstruction and control of a time-dependent two-electron wave packet. Nature, 516, 374(2014).
[4] M. Sabbar, S. Heuser, R. Boge, M. Lucchini, T. Carette, E. Lindroth, L. Gallmann, C. Cirelli, U. Keller. Resonance effects in photoemission time delays. Phys. Rev. Lett., 115, 133001(2015).
[5] M. Ossiander, F. Siegrist, V. Shirvanyan, R. Pazourek, A. Sommer, T. Latka, A. Guggenmos, S. Nagele, J. Feist, J. Burgdörfer, R. Kienberger, M. Schultze. Attosecond correlation dynamics. Nat. Phys., 13, 280(2017).
[6] F. Calegari, D. Ayuso, A. Trabattoni, L. Belshaw, S. De Camillis, S. Anumula, F. Frassetto, L. Poletto, A. Palacios, P. Decleva, J. B. Greenwood, F. Martín, M. Nisoli. Ultrafast electron dynamics in phenylalanine initiated by attosecond pulses. Science, 346, 336(2014).
[7] A. Trabattoni, M. Klinker, J. González-Vázquez, C. Liu, G. Sansone, R. Linguerri, M. Hochlaf, J. Klei, M. J. J. Vrakking, F. Martín, M. Nisoli, F. Calegari. Mapping the dissociative ionization dynamics of molecular nitrogen with attosecond time resolution. Phys. Rev. X, 5, 041053(2015).
[8] M. Huppert, I. Jordan, D. Baykusheva, A. V. Conta, H. J. Wörner. Attosecond delays in molecular photoionization. Phys. Rev. Lett., 117, 093001(2016).
[9] S. Neppl, R. Ernstorfer, A. L. Cavalieri, C. Lemell, G. Wachter, E. Magerl, E. M. Bothschafter, M. Jobst, M. Hofstetter, U. Kleineberg, J. V. Barth, D. Menzel, J. Burgdörfer, P. Feulner, F. Krausz, R. Kienberger. Direct observation of electron propagation and dielectric screening on the atomic length scale. Nature, 517, 342(2015).
[10] Z. Tao, C. Chen, T. Szilvási, M. Keller, M. Mavrikakis, H. Kapteyn, M. Murnane. Direct time-domain observation of attosecond final-state lifetimes in photoemission from solids. Science, 353, 62(2016).
[11] F. Siek, S. Neb, P. Bartz, M. Hensen, C. Strüber, S. Fiechter, M. Torrent-Sucarrat, V. M. Silkin, E. E. Krasovskii, N. M. Kabachnik, S. Fritzsche, R. D. Muiño, P. M. Echenique, A. K. Kazansky, N. Müller, W. Pfeiffer, U. Heinzmann. Angular momentum–induced delays in solid-state photoemission enhanced by intra-atomic interactions. Science, 357, 1274(2017).
[12] R. López-Martens, K. Varjú, P. Johnsson, J. Mauritsson, Y. Mairesse, P. Salières, M. B. Gaarde, K. J. Schafer, A. Persson, S. Svanberg, C. Wahlström, A. L’Huillier. Amplitude and phase control of attosecond light pulses. Phys. Rev. Lett., 94, 033001(2005).
[13] J. P. Wu, Y. H. Zhang, Z. N. Zeng, R. X. Li. High-order harmonic generation from zigzag graphene nanoribbons. Chin. Opt. Lett., 18, 103201(2020).
[14] P. M. Paul, E. S. Toma, P. Breger, G. Mullot, F. Augé, P. Balcou, H. G. Muller, P. Agostini. Observation of a train of attosecond pulses from high harmonic generation. Science, 292, 1689(2001).
[15] T. Remetter, P. Johnsson, J. Mauritsson, K. Varjú, Y. Ni, F. Lépine, E. Gustafsson, M. Kling, J. Khan, R. López-Martens, K. J. Schafer, M. J. J. Vrakking, A. L’Huillier. Attosecond electron wave packet interferometry. Nat. Phys., 2, 323(2006).
[16] G. Sansone, E. Benedetti, F. Calegari, C. Vozzi, L. Avaldi, R. Flammini, L. Poletto, P. Villoresi, C. Altucci, R. Velotta, S. Stagira, S. De Silvestri, M. Nisoli. Isolated single-cycle attosecond pulses. Science, 314, 443(2006).
[17] E. Goulielmakis, M. Schultze, M. Hofstetter, V. S. Yakovlev, J. Gagnon, M. Uiberacker, A. L. Aquila, E. M. Gullikson, D. T. Attwood, R. Kienberger, F. Krausz, U. Kleineberg. Single-cycle nonlinear optics. Science, 320, 1614(2008).
[18] G. C. Li, Y. H. Zheng, Z. N. Zeng, R. X. Li. Intense keV IAP generation by orthogonally polarized multicycle midinfrared two-color laser fields. Chin. Opt. Lett., 15, 071901(2017).
[19] H. G. Muller. Reconstruction of attosecond harmonic beating by interference of two-photon transitions. Appl. Phys. B, 74, s17(2002).
[20] J. Itatani, F. Quéré, G. L. Yudin, M. Y. Ivanov, F. Krausz, P. B. Corkum. Attosecond streak camera. Phys. Rev. Lett., 88, 173903(2002).
[21] M. Schultze, M. Fieß, N. Karpowicz, J. Gagnon, M. Korbman, M. Hofstetter, S. Neppl, A. L. Cavalieri, Y. Komninos, Th. Mercouris, C. A. Nicolaides, R. Pazourek, S. Nagele, J. Feist, J. Burgdörfer, A. M. Azzeer, R. Ernstorfer, R. Kienberger, U. Kleineberg, E. Goulielmakis, F. Krausz, V. S. Yakovlev. Delay in photoemission. Science, 328, 1658(2010).
[22] F. Quéré, J. Itatani, G. L. Yudin, P. B. Corkum. Attosecond spectral shearing interferometry. Phys. Rev. Lett., 90, 073902(2003).
[23] M. Murakami, G. P. Zhang. Observation of attosecond electron dynamics in the photoelectron momentum distribution of atoms using few-cycle laser pulses. Phys. Rev. A, 101, 053439(2020).
[24] J. Su, H. C. Ni, A. Becker, A. Jaron-Bcker. Theoretical analysis of time delays and streaking effects in XUV photoionization. J. Mod. Opt., 60, 1484(2013).
[25] M. Swoboda, J. M. Dahlström, T. Ruchon, P. Johnsson, J. Mauritsson, A. L’Huillier, K. J. Schafer. Intensity dependence of laser-assisted attosecond photoionization spectra. Laser Phys., 19, 1591(2009).
[26] L. Guo, Y. Jia, M. Q. Liu, X. Y. Jia, S. L. Hu, R. H. Lu, S. S. Han, J. Chen. Temporal characterization of electron dynamics in attosecond XUV and infrared laser fields(2021).
[27] L. Cattaneo, J. Vos, M. Lucchini, L. Gallmann, C. Cirelli, U. Keller. Comparison of attosecond streaking and RABBITT. Opt. Express, 24, 29060(2016).
[28] H. R. Reiss. Effect of an intense electromagnetic field on a weakly bound system. Phys. Rev. A, 22, 1786(1980).
[29] L. Guo, M. Q. Liu, R. H. Lu, S. S. Han, J. Chen. Analysis of above-threshold ionization by “Wigner-distribution-like function” method. Laser Part. Beams, 37, 448(2019).
[30] L. Guo, S. S. Han, J. Chen. Time-energy analysis of above-threshold ionization. Opt. Express, 18, 1240(2010).
[31] J. M. Dahlström, A. L’Huillier, A. Maquet. Introduction to attosecond delays in photoionization. J. Phys. B: At. Mol. Opt. Phys., 45, 183001(2012).
[32] K. Varjú, P. Johnsson, J. Mauritsson, T. Remetter, T. Ruchon, Y. Ni, F. Lépine, M. Kling, J. Khan, K. J. Schafer. Angularly resolved electron wave packet interferences. J. Phys. B: At. Mol. Opt. Phys., 39, 3983(2006).
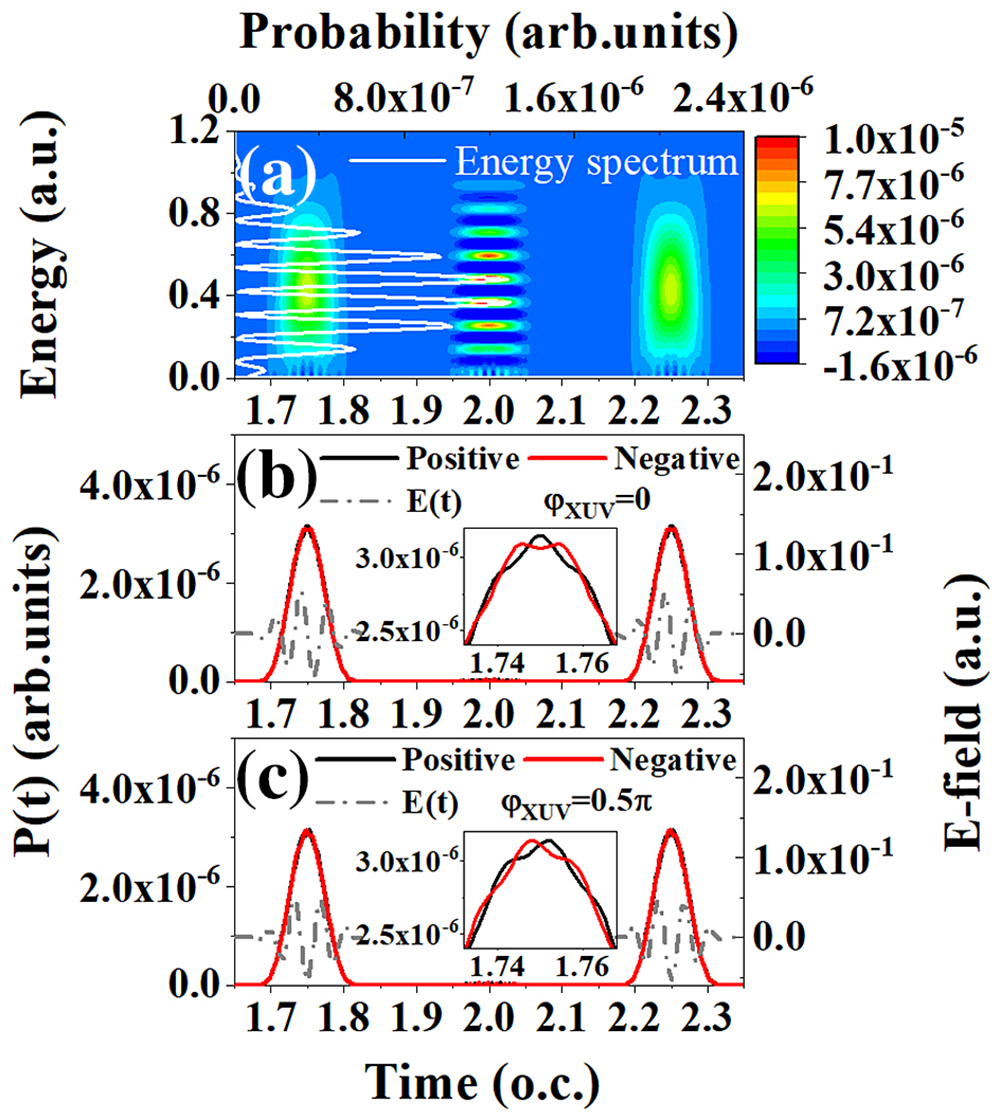
Set citation alerts for the article
Please enter your email address