Xiaoyan Zhou, Liang Zhai, Jin Liu, "Epitaxial quantum dots: a semiconductor launchpad for photonic quantum technologies," Photon. Insights 1, R07 (2022)

Search by keywords or author
- Photonics Insights
- Vol. 1, Issue 2, R07 (2022)
![Quantum dot heterostructures. (a)–(c) Schematic view of quantum dots grown by the Stranski–Krastranov mode, droplet epitaxy, and local droplet etching, respectively. In the Stranski–Krastranov growth mode, the formation of quantum dots is driven by strain relaxation. Quantum dots are placed on a thin two-dimensional wetting layer and exhibit truncated pyramid shapes[50] after capping. In droplet epitaxy, quantum dots crystallize under the flux of group V materials. The quantum-dot shape tends to be more symmetric compared to that of the Stranski–Krastranov mode, and the wetting layer can be suppressed. For local droplet etching, quantum dots are formed by filling shallow nanoholes. The nanoholes are etched by metal droplets (for example, Al); during the etching process, residual materials (for example, AlAs) diffuse and nucleate around the nanohole, forming a ring structure. (d) Sketch of an n-i-p diode structure hosting a quantum dot. Quantum dot confinements in the conduction and valence bands are highlighted in blue and orange, respectively. On application of an external gate voltage Vg, the quantum dot can be tuned into resonance with Fermi sea level, allowing an electron to tunnel into the quantum dot. (e) Photoluminescence map on varying the external gate voltage Vg shows the locking and tuning of the charge states. The abrupt jump from one charge state to another is a sign of a Coulomb blockade. The shift in emission energy in each charge plateau stems from the quantum-confined Stark effect. In this particular quantum dot, 11 charge states, from X2+ to X8−, are mapped out with a spectrometer. (f), (g) Sketches of energy levels in the conduction band where s-, p-, d-shells are visualized. Here, the diode structure is not taken into consideration. In (f), an electron–hole pair is created by aboveband excitation. The electron is captured by the quantum-dot potential, entering different energy levels. When the electron is in the upper excitonic states, such as p- and d-shells, it is unstable and relaxes to the ground state (s-shell) shortly. In the valence band, energy levels can be complicated. For simplicity, only the heavy-hole ground state is shown. In (g), a resonant laser is used to excite the quantum dot, creating an electron–hole pair at the ground states. Panel reproduced with permission from: (e) Ref. [51] under a Creative Commons license CC BY 4.0.](/richHtml/pi/2022/1/2/R07/img_001.png)
Fig. 1. Quantum dot heterostructures. (a)–(c) Schematic view of quantum dots grown by the Stranski–Krastranov mode, droplet epitaxy, and local droplet etching, respectively. In the Stranski–Krastranov growth mode, the formation of quantum dots is driven by strain relaxation. Quantum dots are placed on a thin two-dimensional wetting layer and exhibit truncated pyramid shapes[50] after capping. In droplet epitaxy, quantum dots crystallize under the flux of group V materials. The quantum-dot shape tends to be more symmetric compared to that of the Stranski–Krastranov mode, and the wetting layer can be suppressed. For local droplet etching, quantum dots are formed by filling shallow nanoholes. The nanoholes are etched by metal droplets (for example, Al); during the etching process, residual materials (for example, AlAs) diffuse and nucleate around the nanohole, forming a ring structure. (d) Sketch of an n-i-p diode structure hosting a quantum dot. Quantum dot confinements in the conduction and valence bands are highlighted in blue and orange, respectively. On application of an external gate voltage , the quantum dot can be tuned into resonance with Fermi sea level, allowing an electron to tunnel into the quantum dot. (e) Photoluminescence map on varying the external gate voltage shows the locking and tuning of the charge states. The abrupt jump from one charge state to another is a sign of a Coulomb blockade. The shift in emission energy in each charge plateau stems from the quantum-confined Stark effect. In this particular quantum dot, 11 charge states, from to , are mapped out with a spectrometer. (f), (g) Sketches of energy levels in the conduction band where s-, p-, d-shells are visualized. Here, the diode structure is not taken into consideration. In (f), an electron–hole pair is created by aboveband excitation. The electron is captured by the quantum-dot potential, entering different energy levels. When the electron is in the upper excitonic states, such as p- and d-shells, it is unstable and relaxes to the ground state (s-shell) shortly. In the valence band, energy levels can be complicated. For simplicity, only the heavy-hole ground state is shown. In (g), a resonant laser is used to excite the quantum dot, creating an electron–hole pair at the ground states. Panel reproduced with permission from: (e) Ref. [51] under a Creative Commons license CC BY 4.0 .
![Creation of non-classical light with individual quantum dots. (a)–(d) Schemes to deterministically prepare a quantum dot for a high upper-level population. In (a), the preparation is mediated by a polarized microcavity. In (b), the excitation is performed with dichromatic laser pulses. (c) Alternating between a high and low Rabi frequency sweeps up the excited state population. (d) In a biexciton cascade, an exciton state can be prepared on demand by combining two-photon excitation and a stimulating pulse. (e)–(h) Microstructures and nanostructures designed to increase the collection efficiency of a quantum-dot single-photon source. (e) Microlens microstructure on top of a bottom DBR. (f) Micropillar cavity where the quantum dot sits between two monolithically grown DBR structures. (g) Tunable microcavity in which the top dielectric mirror is free to move in all three dimensions. (h) Nanobeam waveguide that hosts quantum dots. At the two ends of the waveguide, two grating couplers scatter the quantum dot signal (laser light) into (from) the out-of-plane direction. (i)–(k) Using quantum dots to create entangled photons. (i) Quantum dot embedded in a bullseye cavity as a polarization-entangled photon source. (j) Quantum dot converted to a path-entangled photon source by integration into a glide plane-symmetric photonic crystal waveguide. (k) Scheme to create and verify time–bin entangled photon pairs from a quantum-dot biexciton cascade. Panels reproduced with permission from: (a) Ref. [20] Springer Nature Ltd; (b) Ref. [79] Springer Nature Ltd; (c) Ref. [80] APS; (d) Ref. [81] Springer Nature Ltd; (e) Ref. [82] under a Creative Commons license CC BY 4.0; (f) Ref. [20] Springer Nature Ltd; (g) Ref. [21] Springer Nature Ltd; (h) Ref. [83] under a Creative Commons license CC BY 4.0; (i) Ref. [19] Springer Nature Ltd; (j) Ref. [84] APS; (k) Ref. [85] Optica.](/richHtml/pi/2022/1/2/R07/img_002.png)
Fig. 2. Creation of non-classical light with individual quantum dots. (a)–(d) Schemes to deterministically prepare a quantum dot for a high upper-level population. In (a), the preparation is mediated by a polarized microcavity. In (b), the excitation is performed with dichromatic laser pulses. (c) Alternating between a high and low Rabi frequency sweeps up the excited state population. (d) In a biexciton cascade, an exciton state can be prepared on demand by combining two-photon excitation and a stimulating pulse. (e)–(h) Microstructures and nanostructures designed to increase the collection efficiency of a quantum-dot single-photon source. (e) Microlens microstructure on top of a bottom DBR. (f) Micropillar cavity where the quantum dot sits between two monolithically grown DBR structures. (g) Tunable microcavity in which the top dielectric mirror is free to move in all three dimensions. (h) Nanobeam waveguide that hosts quantum dots. At the two ends of the waveguide, two grating couplers scatter the quantum dot signal (laser light) into (from) the out-of-plane direction. (i)–(k) Using quantum dots to create entangled photons. (i) Quantum dot embedded in a bullseye cavity as a polarization-entangled photon source. (j) Quantum dot converted to a path-entangled photon source by integration into a glide plane-symmetric photonic crystal waveguide. (k) Scheme to create and verify time–bin entangled photon pairs from a quantum-dot biexciton cascade. Panels reproduced with permission from: (a) Ref. [20] Springer Nature Ltd; (b) Ref. [79] Springer Nature Ltd; (c) Ref. [80] APS; (d) Ref. [81] Springer Nature Ltd; (e) Ref. [82] under a Creative Commons license CC BY 4.0 ; (f) Ref. [20] Springer Nature Ltd; (g) Ref. [21] Springer Nature Ltd; (h) Ref. [83] under a Creative Commons license CC BY 4.0 ; (i) Ref. [19] Springer Nature Ltd; (j) Ref. [84] APS; (k) Ref. [85] Optica.
![Chiral light–matter interaction. Chiral quantum-dot emission in planar photonic structures, including (a) nanobeam waveguide, (b) waveguide crossing, and (c) photonic crystal waveguide. (d) Single-photon isolator with a quantum dot embedded in an open cavity. Topological quantum photonic interfaces in (e) spin-Hall photonic crystal waveguide and (f) side-coupled triangular-shaped resonator consisting of valley-Hall photonic crystal waveguides. Panels reproduced with permission from: (a) Ref. [109] under a Creative Commons license CC BY 4.0; (b) Ref. [135] AIP Publishing LLC; (c) Ref. [137] Springer Nature Ltd; (d) Ref. [104] under a Creative Commons license CC BY 4.0; (e) Ref. [139] AAAS; (f) Ref. [140] Optica.](/Images/icon/loading.gif)
Fig. 3. Chiral light–matter interaction. Chiral quantum-dot emission in planar photonic structures, including (a) nanobeam waveguide, (b) waveguide crossing, and (c) photonic crystal waveguide. (d) Single-photon isolator with a quantum dot embedded in an open cavity. Topological quantum photonic interfaces in (e) spin-Hall photonic crystal waveguide and (f) side-coupled triangular-shaped resonator consisting of valley-Hall photonic crystal waveguides. Panels reproduced with permission from: (a) Ref. [109] under a Creative Commons license CC BY 4.0 ; (b) Ref. [135] AIP Publishing LLC; (c) Ref. [137] Springer Nature Ltd; (d) Ref. [104] under a Creative Commons license CC BY 4.0 ; (e) Ref. [139] AAAS; (f) Ref. [140] Optica.
![Single-photon nonlinearity. (a) A quantum dot strongly coupled to a photonic crystal cavity can work as an ultrafast single-photon switch. (b) A quantum dot in a photonic crystal waveguide works effectively as a single-photon transistor. The single-photon component gets reflected while the two-photon and higher-photon components are transmitted. HBT measurement performed on reflected light using a very weak resonant laser as the input reveals photon antibunching: the reflection has a single-photon character. In transmission, the experiment performed similarly shows photon bunching: the transmission has a multi-photon character. (c) A quantum dot coupled to a micropillar shows a greatly suppressed three-photon component in the reflection. It can be viewed as a filter that removes multi-photon components from single photons. (d) Photon–photon interaction dynamics in a quantum-dot–PhC waveguide system. Panels reproduced with permission from: (a) Ref. [23] Springer Nature Ltd; (b) Ref. [24] under a Creative Commons license CC BY 4.0; (c) Ref. [144] Springer Nature Ltd; (d) Ref. [159] Springer Nature Ltd.](/Images/icon/loading.gif)
Fig. 4. Single-photon nonlinearity. (a) A quantum dot strongly coupled to a photonic crystal cavity can work as an ultrafast single-photon switch. (b) A quantum dot in a photonic crystal waveguide works effectively as a single-photon transistor. The single-photon component gets reflected while the two-photon and higher-photon components are transmitted. HBT measurement performed on reflected light using a very weak resonant laser as the input reveals photon antibunching: the reflection has a single-photon character. In transmission, the experiment performed similarly shows photon bunching: the transmission has a multi-photon character. (c) A quantum dot coupled to a micropillar shows a greatly suppressed three-photon component in the reflection. It can be viewed as a filter that removes multi-photon components from single photons. (d) Photon–photon interaction dynamics in a quantum-dot–PhC waveguide system. Panels reproduced with permission from: (a) Ref. [23] Springer Nature Ltd; (b) Ref. [24] under a Creative Commons license CC BY 4.0 ; (c) Ref. [144] Springer Nature Ltd; (d) Ref. [159] Springer Nature Ltd.
![Spins trapped in quantum dots and a spin–photon interface. (a) Optical means to manipulate a single electron spin trapped in a quantum dot. With an in-plane magnetic field, the trion state forms a four-level system. Typically, two vertical and two diagonal transitions are orthogonal in polarization and similar in oscillator strength. The spin states, |↑〉, |↓〉, and their superposition, can be initialized and read out using an optical pulse that is resonant to one of four transitions. Employing a far detuned pulsed laser allows for coherently rotating the spin state. As a function of the power of this rotation laser, a Rabi oscillation between two spin ground states is revealed. (b) Scheme for cooling the nuclear spins surrounding the quantum dot, which reduces the nuclear spin fluctuation, thereby increasing the coherence time T2* of the electron spin. (c) The principal electron spin resonance and its four sidebands are visible when the nuclear spin ensemble is polarized to a state with a very narrow probability distribution. By selectively choosing two-photon detuning, a single nuclear spin can be excited from the nuclear-spin ensemble. (d) Distant spins trapped in two quantum dots are entangled using Raman-scattered photons and an optical interferometer. Joint spin correlations in the spin basis and the rotating basis defined by the superposition are shown in the bottom panel, yielding an average entanglement fidelity of around 62%. Orange: joint spin projection in the population basis; red: projection in the rotated basis of a potential |Ψ+〉-state; blue: rotated basis, potentially a |Ψ−〉-state. Panels reproduced with permission from: (a) Ref. [64] under a Creative Commons license CC BY 4.0 and Ref. [166] Springer Nature Ltd; (b) Ref. [62] APS; (c) Ref. [63] AAAS; (d) Ref. [167] APS.](/Images/icon/loading.gif)
Fig. 5. Spins trapped in quantum dots and a spin–photon interface. (a) Optical means to manipulate a single electron spin trapped in a quantum dot. With an in-plane magnetic field, the trion state forms a four-level system. Typically, two vertical and two diagonal transitions are orthogonal in polarization and similar in oscillator strength. The spin states, , , and their superposition, can be initialized and read out using an optical pulse that is resonant to one of four transitions. Employing a far detuned pulsed laser allows for coherently rotating the spin state. As a function of the power of this rotation laser, a Rabi oscillation between two spin ground states is revealed. (b) Scheme for cooling the nuclear spins surrounding the quantum dot, which reduces the nuclear spin fluctuation, thereby increasing the coherence time of the electron spin. (c) The principal electron spin resonance and its four sidebands are visible when the nuclear spin ensemble is polarized to a state with a very narrow probability distribution. By selectively choosing two-photon detuning, a single nuclear spin can be excited from the nuclear-spin ensemble. (d) Distant spins trapped in two quantum dots are entangled using Raman-scattered photons and an optical interferometer. Joint spin correlations in the spin basis and the rotating basis defined by the superposition are shown in the bottom panel, yielding an average entanglement fidelity of around 62%. Orange: joint spin projection in the population basis; red: projection in the rotated basis of a potential -state; blue: rotated basis, potentially a -state. Panels reproduced with permission from: (a) Ref. [64] under a Creative Commons license CC BY 4.0 and Ref. [166] Springer Nature Ltd; (b) Ref. [62] APS; (c) Ref. [63] AAAS; (d) Ref. [167] APS.
![Recent advances in reconfigurable quantum circuits. (a)–(d) Quantum dots integrated with single-photon routers. (a) An array of quantum dots in an electric diode structure (electroluminescence) is bonded and butt-coupled to a thermo-optically tunable silicon oxynitride QPIC consisting of phase shifters and switches in the form of MZIs. (b), (c) Monolithic integration of quantum dots with photonic switches on GaAs platform. The switch is realized by an electro-optically tunable MZI in (b) and a compact directional coupler (DC) reconfigured by a nano-opto-mechanical system (NOEMS) in (c). (d) Hybrid integration of quantum dots grown in a nanowire with thermo-optically tunable SiN microring filters/switches. (e)–(h) Hybrid quantum photonic platforms, where quantum dots are integrated with (e) LiNbO3 waveguide and (f) Si waveguide using the pick-and-place method, with (g) GaAs waveguide using the transfer-printing method, and (h) SiN waveguide using wafer bonding. (i)–(l) State-of-the-art switches and modulators in general. (i) Cryogenic-compatible reconfigurable LiNbO3 switch of gigahertz bandwidth integrated with on-chip single-photon detector. (j) Cryogenic-compatible piezo-optomechanical MZI switches on a hybrid AlN-SiN platform. (k) Reconfigurable NOEMS switch consisting of SiN DCs and integrated with cryogenic single-photon detector. (l) Large-scale and broadband NOEMS-actuated Si digital (or on–off) switches based on vertical waveguide couplers. Panels reproduced with permission from: (a) Ref. [33] AIP Publishing LLC; (b) Ref. [202] Optica; (c) Ref. [34] Optica; (d) Ref. [32] under a Creative Commons license CC BY 4.0; (e) Ref. [203] AIP Publishing LLC; (f) Ref. [204] ACS; (g) Ref. [205] Optica; (h) Ref. [206] under a Creative Commons license CC BY 4.0; (i) Ref. [207] under a Creative Commons license CC BY 4.0; (j) Ref. [208] Springer Nature Ltd; (k) Ref. [209] under a Creative Commons license CC BY 4.0; (l) Ref. [210] Optica.](/Images/icon/loading.gif)
Fig. 6. Recent advances in reconfigurable quantum circuits. (a)–(d) Quantum dots integrated with single-photon routers. (a) An array of quantum dots in an electric diode structure (electroluminescence) is bonded and butt-coupled to a thermo-optically tunable silicon oxynitride QPIC consisting of phase shifters and switches in the form of MZIs. (b), (c) Monolithic integration of quantum dots with photonic switches on GaAs platform. The switch is realized by an electro-optically tunable MZI in (b) and a compact directional coupler (DC) reconfigured by a nano-opto-mechanical system (NOEMS) in (c). (d) Hybrid integration of quantum dots grown in a nanowire with thermo-optically tunable SiN microring filters/switches. (e)–(h) Hybrid quantum photonic platforms, where quantum dots are integrated with (e) waveguide and (f) Si waveguide using the pick-and-place method, with (g) GaAs waveguide using the transfer-printing method, and (h) SiN waveguide using wafer bonding. (i)–(l) State-of-the-art switches and modulators in general. (i) Cryogenic-compatible reconfigurable switch of gigahertz bandwidth integrated with on-chip single-photon detector. (j) Cryogenic-compatible piezo-optomechanical MZI switches on a hybrid AlN-SiN platform. (k) Reconfigurable NOEMS switch consisting of SiN DCs and integrated with cryogenic single-photon detector. (l) Large-scale and broadband NOEMS-actuated Si digital (or on–off) switches based on vertical waveguide couplers. Panels reproduced with permission from: (a) Ref. [33] AIP Publishing LLC; (b) Ref. [202] Optica; (c) Ref. [34] Optica; (d) Ref. [32] under a Creative Commons license CC BY 4.0 ; (e) Ref. [203] AIP Publishing LLC; (f) Ref. [204] ACS; (g) Ref. [205] Optica; (h) Ref. [206] under a Creative Commons license CC BY 4.0 ; (i) Ref. [207] under a Creative Commons license CC BY 4.0 ; (j) Ref. [208] Springer Nature Ltd; (k) Ref. [209] under a Creative Commons license CC BY 4.0 ; (l) Ref. [210] Optica.
![Applications of quantum dots in quantum networks. (a) Entanglement swapping between two photonic entangled pairs created by quantum-dot biexciton cascade. (b) Quantum cryptography is performed using quantum-dot entangled photons and the BBM92 protocol. The key is distributed between two buildings that are 350 m apart over a fiber link. (c) Scheme of device-independent QKD that relies on single-photon sources and central heralding instead of entangled photon-pair generation. The scheme promises great improvement in attainable key rates and communication distances. (d) Single photons created by two separate quantum dots remain indistinguishable. In a Hong–Ou–Mandel-type experiment performed with photons from two separate quantum dots, the coincidence events in the zero-delayed peak account for around 7% compared to that in the classical case, equivalently, Hong–Ou–Mandel visibility of 93%. (e) Emission spectrum and radiative time measurements of a quantum dot close to telecom C-band. (f) Sending photon emission from a GaAs quantum dot through a rubidium vapor cell shows clear absorption at the rubidium D2 line. Panels reproduced with permission from: (a) Ref. [274] APS; (b) Ref. [280] Chinese Laser Press; (c) Ref. [282] under a Creative Commons license CC BY 4.0; (d) Ref. [57] Springer Nature Ltd; (e) Ref. [283] under a Creative Commons license CC BY 4.0; (f) Ref. [284] Springer Nature Ltd.](/Images/icon/loading.gif)
Fig. 7. Applications of quantum dots in quantum networks. (a) Entanglement swapping between two photonic entangled pairs created by quantum-dot biexciton cascade. (b) Quantum cryptography is performed using quantum-dot entangled photons and the BBM92 protocol. The key is distributed between two buildings that are 350 m apart over a fiber link. (c) Scheme of device-independent QKD that relies on single-photon sources and central heralding instead of entangled photon-pair generation. The scheme promises great improvement in attainable key rates and communication distances. (d) Single photons created by two separate quantum dots remain indistinguishable. In a Hong–Ou–Mandel-type experiment performed with photons from two separate quantum dots, the coincidence events in the zero-delayed peak account for around 7% compared to that in the classical case, equivalently, Hong–Ou–Mandel visibility of 93%. (e) Emission spectrum and radiative time measurements of a quantum dot close to telecom C-band. (f) Sending photon emission from a GaAs quantum dot through a rubidium vapor cell shows clear absorption at the rubidium line. Panels reproduced with permission from: (a) Ref. [274] APS; (b) Ref. [280] Chinese Laser Press; (c) Ref. [282] under a Creative Commons license CC BY 4.0 ; (d) Ref. [57] Springer Nature Ltd; (e) Ref. [283] under a Creative Commons license CC BY 4.0 ; (f) Ref. [284] Springer Nature Ltd.
![Quantum dot for quantum information processing. (a) Boson sampling with 20 highly indistinguishable single photons as input towards demonstration of quantum advantage. The spatially parallel photons are generated from a demultiplexed InGaAs quantum dot embedded in a micropillar cavity. (b) Quantum simulation of four-atom molecular vibration dynamics with four single photons, and proof-of-concept experiment with single photons produced from spontaneous parametric downconversion sources. (c) Heralded non-destructive Bell-state generation from four distinguishable single photons, which are demultiplexed from a quantum-dot single-photon source similar to (a). (d) Deterministic generation of time–bin entangled photons by coherently manipulating the hole–spin of a quantum dot in a photonic crystal waveguide. (e) Proposal for deterministic generation of a 2D photonic cluster state by routing time-delayed 1D cluster generated from a quantum dot back to itself. (f) Proposal for direct 2D cluster state generation with coupled quantum dots. Panels reproduced with permission from: (a) Ref. [118] APS; (b) Ref. [330] Springer Nature Ltd; (c) Ref. [337] APS; (d) Ref. [338] APS; (e) Ref. [339] NAS; (f) Ref. [340] APS.](/Images/icon/loading.gif)
Fig. 8. Quantum dot for quantum information processing. (a) Boson sampling with 20 highly indistinguishable single photons as input towards demonstration of quantum advantage. The spatially parallel photons are generated from a demultiplexed InGaAs quantum dot embedded in a micropillar cavity. (b) Quantum simulation of four-atom molecular vibration dynamics with four single photons, and proof-of-concept experiment with single photons produced from spontaneous parametric downconversion sources. (c) Heralded non-destructive Bell-state generation from four distinguishable single photons, which are demultiplexed from a quantum-dot single-photon source similar to (a). (d) Deterministic generation of time–bin entangled photons by coherently manipulating the hole–spin of a quantum dot in a photonic crystal waveguide. (e) Proposal for deterministic generation of a 2D photonic cluster state by routing time-delayed 1D cluster generated from a quantum dot back to itself. (f) Proposal for direct 2D cluster state generation with coupled quantum dots. Panels reproduced with permission from: (a) Ref. [118] APS; (b) Ref. [330] Springer Nature Ltd; (c) Ref. [337] APS; (d) Ref. [338] APS; (e) Ref. [339] NAS; (f) Ref. [340] APS.
|
Table 1. State-of-the-Art Performance of a Photonic Switch in Various Material Platforms.
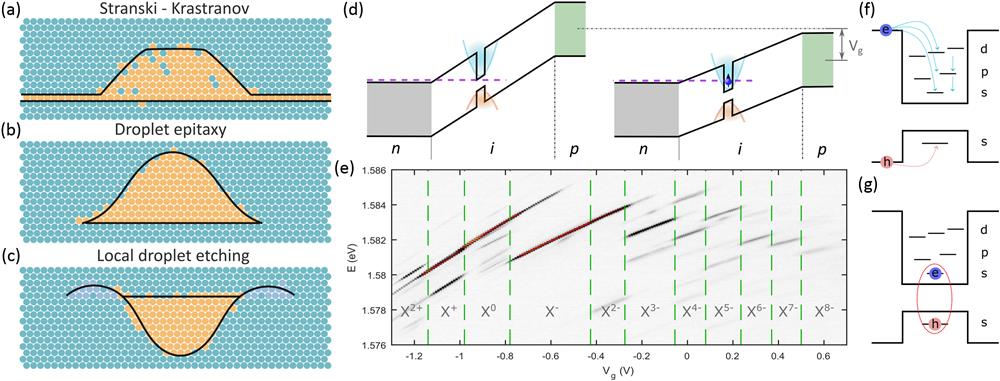
Set citation alerts for the article
Please enter your email address