
- Photonics Research
- Vol. 9, Issue 3, 289 (2021)
Abstract
1. INTRODUCTION
Soliton collisions, as one of the most fascinating interactions in nonlinear systems, have been extensively investigated in the fields of metamaterials [1], photorefractive materials [2,3], Bose–Einstein condensates [4,5], and fiber optics [6,7]. In the framework of the nonlinear Schrödinger equation, due to their particle-like features, scalar solitons collide only by phase and position shifts with unaltered shape, amplitude, or speed after passing through one another [4,6]. As a remarkable contrast, collision interactions between vector solitons exhibit richer phenomena, such as intensity redistributions [8,9], energy-exchange interactions [2], and fractal structure [10]. Such wealthy nonlinear dynamics in collision of vector solitons paves the way for potential applications in all-optical logic and universal computation [11,12].
Optical fiber is an excellent platform for investigating the propagation and collision of vector solitons. To date, extensive theoretical and numerical but few experimental investigations have been reported to give insight into the intrinsic nature of the vector-soliton collision dynamics [6,8,10,13,14]. The first experimental observation of temporal vector-soliton collision was demonstrated in a linearly birefringent fiber, in which an intensity redistribution between both polarization components was observed before and after collision [6]. Nevertheless, visualized mutual spectral–temporal evolutions of both vector solitons during collision were not referenced. In addition, stable vector solitons in such a conservative system require only the balance between fiber nonlinearity and dispersion. Dissipative systems, such as mode-locked lasers, involve more rigorous requirements with another balance between gain and loss. Therefore, collision dynamics of vector solitons might characterize more marvelous features in mode-locked lasers than in birefringent fibers. More recent reports have shown that vector solitons with different group velocities can be steadily operated in each polarization component of optical fibers by inserting a segment of polarization-maintaining fiber (PMF) in a mode-locked fiber laser [15]. The slightly different repetition rates of both vector solitons lead to their mutual collisions from time to time. Although this fiber laser can act as an ideal candidate to investigate vector-soliton collisions, it remains unclear about the detailed collision dynamics so far.
The fast transient dynamics of soliton collisions in fiber lasers cannot be retrieved by conventional averaged optical spectral analyzers (OSAs) and autocorrelators, due to the limited measurement bandwidth. More recently, the time-stretch dispersive Fourier transform (TS-DFT) technique, developed for real-time spectral characterization, has been adopted to reveal the buildup and internal dynamics of soliton molecules [16–19], the behaviors of soliton buildup [20–22], and exotic dynamics of soliton explosions [23–26]. This technique has been demonstrated as a powerful tool for the measurement of rapid scalar soliton-collision processes [27,28]. Based on this excellent technique, we systematically investigated the buildup dynamics of asynchronous vector solitons in fiber lasers in our preliminary work [29].
Sign up for Photonics Research TOC. Get the latest issue of Photonics Research delivered right to you!Sign up now
Here, we experimentally and numerically investigate, with the help of the TS-DFT technique, the single-shot dynamics of vector-soliton collisions in a polarization-multiplexed mode-locked fiber laser. Before collision, another two weak pulses are formed by cross-polarization coupling between the orthogonal polarization components. When overlapping with each other, both vector solitons experience opposite central wavelength shifts induced by cross-phase modulation (XPM). A distinct intensity difference in the weak pulse before and after collision implies intensity redistribution. Moreover, the non-conservative total energy of both vector solitons occurs during the collision process. Eventually, both solitons recover to their initial states after several hundred roundtrip (RT) evolutions. Under specific conditions, extreme collisions with strong four-wave-mixing (FWM) sidebands are observed by virtue of coherent coupling, which extends the scenario of FWM sideband generation from steady mode locking to transient collisions. Our study might not only provide deep insight into the collision dynamics of vector solitons, but also contribute to improving the laser design for its application prospect in dual-comb spectroscopy [30–32].
2. GENERATION OF VECTOR SOLITONS WITH DIFFERENT REPETITION RATES
Figure 1.Generation and characterization of two vector solitons with slightly different repetition rates from a mode-locked fiber laser. (a) Schematic of the mode-locked fiber laser (blue) and single-shot spectral measurement (orange). WDM, wavelength-division multiplexer; EDF, erbium-doped fiber; ISO, isolator; CNT-SA, carbon-nanotube saturable absorber; PMF, polarization-maintaining fiber; PC, polarization controller; PBS, polarization beam splitter; SMF, single-mode fiber; EDFA, erbium-doped fiber amplifier. (b), (c) Optical and radio frequency spectra of the two vector solitons before (black) and after (red and blue) passing through PBS. (d), (e) Stable single-shot spectra of the two vector solitons before collisions.
3. EXPERIMENTAL OBSERVATIONS
A. Moderate Collisions
Figure 2.Experimental real-time characterization of moderate collision of vector solitons. (a) Single-shot collision dynamics of vector solitons by the TS-DFT technique. (b), (c) Close-up of the data in (a) before, after, and during collision, respectively. (d), (f) Real-time spectral evolutions of the two vector solitons during collisions. (e), (g) Close-up of the data in (d), (f), respectively. (h) Field autocorrelation trace in (f) via Fourier transform. (i) Close-up of the data in (h). (j) Central wavelength evolutions of vector solitons (d), (f) during collisions. (k) Energies of both vector solitons (red and blue) and their total energy (black).
We then plot their single-shot spectral interferograms and Fourier transformed field autocorrelations over 3000 RTs [Figs. 2(d), 2(f), and 2(h)] to reveal the universal dynamics of vector-soliton collisions. Figures 2(e), 2(g), and 2(i), including the most representative colliding information, are the enlarged views of Figs. 2(d), 2(f), and 2(h), respectively, from 1630th to 1800th RTs. Due to its shorter central wavelength, broader spectral bandwidth, and higher repetition rate [Figs. 1(b) and 1(c)], the vector soliton in Fig. 2(e) (horizontal polarization) holds faster moving speed than the other one [vertical polarization in Fig. 2(g)]. In this scenario, the horizontally polarized vector soliton catches up with and passes through the vertically polarized one. During the 1630th to 1660th RTs, dense spectral interference patterns of both vector solitons indicate the appearance of a weak pulse at each polarization component (these weak pulses are formed by cross-polarization coupling between the orthogonal polarization components in Section 4), as evidence of two weak peaks symmetrically located on both sides of an intense peak from the field autocorrelation trace in Fig. 2(i). Note that the 0.14 nm spectral resolution of our DFT system determines its recording limitation of pulse separation of
The collision position is at the 1666th RT. Remarkably, there are both suddenly opposite central wavelength shifts from 1666th to 1667th RTs, ascribed to the exchange of their overlapped parts, i.e., the trailing and leading edges of the horizontally and vertically polarized vector solitons, respectively, are overlapped after collision. Predominant shifts of the fringe patterns from red to blue and gradually slowing down can be observed during the 1667th to 1800th RTs, representing monotonous changes and tendency to an extremum of the relative phase between the weak pulse and identically polarized vector soliton [33]. Also, the denser and denser spectral interference patterns [Figs. 2(e) and 2(g)] correspond to their larger and larger temporal separations [Fig. 2(i)]. After collision, the speed of walking off between both vector solitons is slower than that before, and experiences the processes of acceleration and rapid recovering. Similar to soliton trapping, the two orthogonally polarized vector solitons shift their central wavelengths in opposite directions, as depicted in Fig. 2(j). The close central wavelengths decrease their group velocity difference, leading to stronger soliton–soliton interactions induced by XPM. Eventually, gradually reduced interactions along with their increased temporal separation result in the recovery, including the central wavelengths and group velocity difference.
Figure 3.Experimental real-time characterization of extreme collision of vector solitons. (a) Single-shot collision dynamics of vector solitons by the TS-DFT technique. (b) Close-up of the data in (a) after collision. (c), (e) Real-time spectral evolution of the two vector solitons during collisions. (d), (f) Close-up of the data in (c) and (e), respectively. (g) Field autocorrelation trace in (e) via Fourier transform. (h) Close-up of the data in (g).
Figure 4.Numerical simulations of moderate collision of vector solitons. (a1), (b1), (e1) Spectral evolution, close-up of the data in (a1), and Kelly sideband rebuilding process of vector soliton in fast axis, respectively. (a2), (b2), (e2) Corresponding spectral evolution, close-up of the data in (a2), and Kelly sideband rebuilding process of vector soliton in slow axis, respectively. (c), (d), (f) Temporal evolution, field autocorrelation trace, and dispersive wave shedding of vector soliton in fast axis, respectively. (g1), (g2) Energy evolutions of vector solitons in fast (red) and slow (blue) axes, and total energy evolution. Inset: close-up of the data in (c).
B. Extreme Collisions
As depicted in Fig. 3(a), extreme collisions, a mass of intense peaks locating on a vector soliton after collision (55–75 μs), occur when properly tuning the PC in the laser cavity. A zoom-in of the extremely colliding region (63.95–64.05 μs) is shown in Fig. 3(b). Obviously, narrow peaks with unequal intensities appear only on the vertically polarized vector soliton, which features stronger spectral peak power and narrower spectral bandwidth than the other one. It is worth noting that the narrow peaks, propagating together with the vector soliton, are coherent components rather than continuous-wave emission; otherwise, they would disperse and disappear after TS-DFT [34].
Analogous to the moderate collision, the entire spectral evolutions of extreme collisions in Figs. 3(c) and 3(e) include stable mode locking before collision, gradually and suddenly opposite central wavelength shifts, a gradual recovery process, and recovered stable mode locking after collision. The differences are the nearly periodic narrow peaks during the recovery process [Fig. 3(f)], with a period of 15 RTs, in accordance with its periodic intensity field autocorrelation trace in Fig. 3(h). As a contrast, another polarized vector soliton presents a smooth evolution without any tendency of narrow peaks in Fig. 3(d).The detailed explanations are explored in Section 4.
4. NUMERICAL SIMULATIONS
A. Moderate Collisions
To give more insight into the collision dynamics of vector solitons in mode-locked fiber lasers, the split-step Fourier method is adopted to solve the coupled Ginzburg–Landau equations in a lumped propagation model [35] (see Appendix B). Numerical simulations start from two weak pulses located at the fast and slow axes of the cavity, with a temporal interval of 280 ps, to speed up the convergence of our simulations. Both pulses converge into their stable solutions after 400 RT circulations. The initial front pulse is located at the fast axis; thus, the two pulses are close to each other and collide at the
The temporal evolutions of both vector solitons during collision, which cannot be measured directly by the TS-DFT technique, provide further insight into the collision dynamics. The collision position is at the 150th RT, as illustrated in Fig. 4(c), of temporal evolution of the vector soliton in the fast axis.
Remarkably, temporal shifts from the 149th to 150th RTs move faster than before [inset in Fig. 4(c)], which could be attributed to the increased group velocity difference between vector solitons induced by opposite spectral shifts [Figs. 4(b1) and 4(b2)]. After collision, another pulse clearly appears at each polarization component. Actually, the weak pulse has also existed before collision, but is not shown in Fig. 4(c) due to its weak intensity. To better show it, we plot the fast axis field autocorrelation trace and tune the upper limit of the colorbar, as sketched in Fig. 4(d). Simulations show that the weak pulse, trapping another axis vector soliton to propagate together, is stable before collision, indicating an incomplete coincidence between polarization directions of the vector solitons and principal axes of the fibers. To explore whether the weak pulse is induced by the dominant soliton through XPM, we deliberately remove the XPM terms in our simulations. It is noticed that the weak pulse continuously damps and eventually disappears in this case. When re-adding the XPM terms, the weak pulse gradually arises and stabilizes again, indicating that the induced weak pulse is formed by cross-polarization coupling between the orthogonal polarization components [36]. A distinct intensity difference in the weak pulse before and after collision [Fig. 4(d)] implies intensity redistribution [2,8,9].
As was expected, vector-soliton collisions also result in dispersive wave shedding, as depicted in Fig. 4(f). Both the dominant soliton and weak pulse symmetrically shed some energy in the form of collision-induced dispersive waves, leading to complicated patterns between them due to the superposition of dispersive waves. Subsequently, the dispersive waves are broadened and weakened by fiber dispersion during circulation in the cavity. Far away from each other between the dominant soliton and weak pulse, the dispersive wave shedding becomes weaker and weaker and gradually disappears for recovery to the initial state before collision. The energy evolutions in Figs. 4(g1) and 4(g2) also match well with the experiments [Fig. 2(k)].
B. Extreme Collisions
Figure 5.Numerical simulations of extreme collision of vector solitons. (a1), (a2) Spectral evolutions of the two vector solitons during collisions. (b1), (b2) Close-up of the data in (a1), (a2), respectively. (c1), (c2) Optical spectra at the 498th RT. (d1), (d2) Temporal traces at the 498th RT. (e1), (e2) Optical spectra of the dominant soliton [red curves, corresponding to the temporal traces in red boxes in (d)] and weak pulse [blue curves, corresponding to the temporal traces in blue boxes in (d)] by Fourier transform. (f1), (f2) Spectral evolutions of the dominant soliton and weak pulse, respectively, from the 490th to 500th RTs in (a2). Insets in (b2): close-up of the spectral evolutions (upper right) and temporal evolutions of the weak pulse (bottom right) from the 300th to 320th RTs.
Both experiments [Fig. 3(f)] and simulations [Figs. 5(b2) and 5(f2)] present the quasi-periodic intensity evolutions of the FWM sideband. The temporal evolutions of the weak pulse in the slow axis [bottom right inset in Fig. 5(b2)] behave analogously to soliton pulsation, which has been extensively investigated in mode-locked lasers [40–42]. Given that, regardless of the negligible intensity change in the dominant soliton, the coherent coupling term [Eq. (B1) in Appendix B] is proportional to the electric field amplitude, and the quasi-periodic FWM sidebands can be traced to the quasi-periodic intensity variations of the weak pulse.
5. DISCUSSION AND CONCLUSION
A mode-locked fiber laser, as a complicated nonlinear dissipative system, provides a unique opportunity for exploring rich interactions between solitons. Our polarization-multiplexed mode-locked fiber laser with strong and weak birefringence in PMF and single-mode fiber (SMF), respectively, could be considered as a birefringence-managed fiber cavity within periodic gain and loss. Strong birefringence in PMF facilitates the generation of close-to-orthogonal vector solitons with slightly different repetition rates. The non-orthogonal polarization between both vector solitons could lead to cross-talk in each polarization. The 30 dB PER in Fig. 1(c) indicates that there could be 0.1% power of the dominant pulse located on the orthogonally polarized axis. Numerical simulations in Figs. 5(d1) and 5(d2) show that the intensity of the weak pulse in the slow axis is about 1.5% of the dominant pulse in the fast axis, indicating that there is little influence of cross-talk on the physical mechanism of the generated weak pulse. In addition, numerical simulations match well with the experiments, indirectly indicating little influence. The longer the PMF utilized in the fiber cavity, the larger the repetition rate difference between vector solitons. Both a small repetition rate difference (217 Hz in our experiments) and weak birefringence in SMF extend the interaction length between vector solitons. According to Ref. [27], the interaction length between vector solitons in our experiments could be approximated to 34.5 m, corresponding to an interaction time of 172 ns. Such a long soliton mutual interaction time enhances the nonlinear phase accumulation of both vector solitons during collision, inducing fascinating moderate and extreme collision dynamics. Extreme collision with FWM sidebands can be derived from the phase matching of both polarization components, which is strictly dependent on the net cavity birefringence. By properly adjusting the net cavity birefringence through the PC, both polarization components of the dominant vector soliton and the weak pulse might satisfy the phase-matching condition, leading to the generation of FWM sidebands. When far away from the phase-matching condition by tuning the PC, the collision dynamics presents moderate collisions. Compared to the vector soliton collision in a conservative system [6], degenerate FWM induced by the coherent coupling term in Eq. (B1) must be retained due to the sub-picosecond soliton pulses and dominant low birefringence in our fiber laser, leading to peculiar FWM spectral sidebands in an extreme collision process. When increasing the cavity length to dozens of meters or even more, the FWM sideband predictably disappears during vector soliton collisions, which we will investigate in future work. Moreover, temporal intensity and central wavelength in each soliton recover to their original status after collision due to the self-consistent conditions of the laser cavity, as shown in Appendix C.
It deserves to be mentioned that FWM spectral sidebands were observed only in steady operations of vector solitons in weakly birefringent mode-locked fiber lasers [38,39]. Our new findings of vector-soliton-collision-induced generation of FWM spectral sidebands could extend the dynamics of soliton–soliton interactions. Such transient dynamics cannot be resolved by conventional OSA, indicating the powerful ability of the TS-DFT technique in investigations of real-time spectral evolutions of dissipative solitons.
Our polarization-multiplexed mode-locked fiber laser can generate vector solitons with slightly different repetition rates, which has potential application in dual-comb spectroscopy. The configuration of dual-comb spectroscopy often consists of signal and reference arms, and the normalized transmission of the sample can be extracted by comparing the signal and reference spectra with heterodyne detections [43]. If considering the ideal case of the same collision process between both vector solitons during each collision in the fiber laser, the disturbances of their transient optical spectra induced by soliton collisions do not influence the measurement precision because of the approach of calibrating the real-time reference spectra. However, each collision process cannot be ensured to be absolutely the same, due to the environment perturbation, such that the signal-to-noise ratio of the measurement might decrease when adopting a coherent average. This could be resolved by several approaches, such as the real-time detection of repetition rate of the interferogram or correction algorithm [32].
In conclusion, we have explored the real-time moderate and extreme collision dynamics of vector solitons by the TS-DFT technique in a polarization-multiplexed mode-locked fiber laser. Cross-polarization coupling between the orthogonal polarization components of vector solitons induces another two weak pulses, and they experience distinct intensity redistribution after collision. Opposite central wavelength shifts, a gradual recovery process, and eventually recovering to their initial states of both vector solitons occur during collision. Furthermore, coherent coupling between the orthogonal polarization components of vector solitons can also lead to strong FWM sidebands. We believe that our results not only provide new perspectives in the collision dynamics of vector solitons in dissipative systems, but also pave the way towards the physical understanding of complex nonlinear science.
Acknowledgment
Acknowledgment. The authors are grateful for the fruitful discussions with Xiaoming Qiu (Peking University) and Frank Wise (Cornell University) on this paper.
APPENDIX A: EXPERIMENTAL SETUP
A 0.5?m erbium-doped fiber (EDF, LEKKI Er110-4/125) is backward-pumped by a 980?nm laser diode via a wavelength-division multiplexer (WDM). The saturable absorber (SA) of a carbon nanotube (CNT) is adopted in the laser cavity to assist mode locking. A polarization-independent isolator (ISO) ensures the unidirectional operation of the laser, and an optical coupler extracts 20% intracavity power for measurement. The most important part of the cavity is a 0.2?m piece of PMF with a beat length of
The single-shot spectral measurement based on the TS-DFT technique consists of a
APPENDIX B: NUMERICAL SIMULATION MODEL
To give more insight into the collision dynamics of vector solitons in mode-locked fiber lasers, the split-step Fourier method is adopted to solve the coupled Ginzburg–Landau equations in a lumped propagation model [
Parameters Used in the Simulations
OC | SA | SMF | PMF | EDF |
---|---|---|---|---|
Gaussian shape |
APPENDIX C: ENERGY REDISTRIBUTIONS IN MODERATE COLLISIONS
Reference [
Figure 6.Numerical simulation results of (a), (b) total energy in each soliton and in each component, (c) temporal intensity, and (d) central wavelength in each soliton.
References
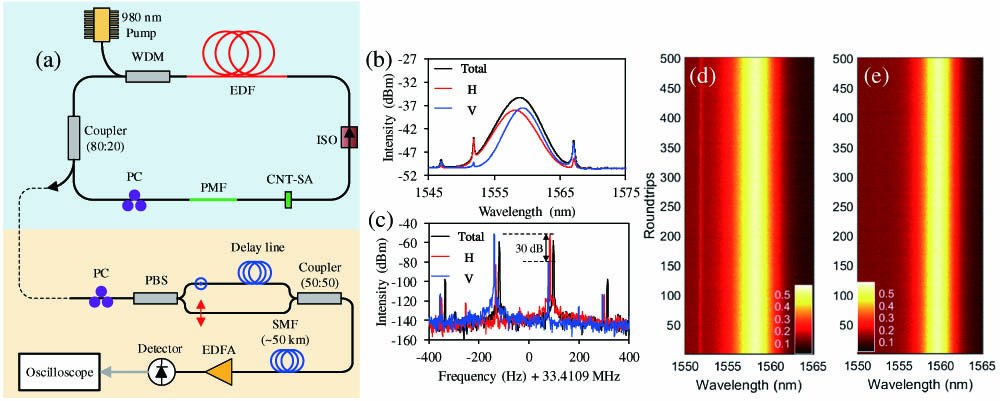
Set citation alerts for the article
Please enter your email address