
- Chinese Optics Letters
- Vol. 20, Issue 6, 061401 (2022)
Abstract
Keywords
1. Introduction
Tunable diode laser absorption spectroscopy (TDLAS) technology utilizes the narrow linewidth and wavelength tunability of semiconductor lasers to realize gas detection in real time and has the advantages of noncontact, high resolution, high sensitivity, and fast response time[
Sign up for Chinese Optics Letters TOC. Get the latest issue of Chinese Optics Letters delivered right to you!Sign up now
Methane gas molecules have absorption lines in both near-infrared and mid-infrared bands. The absorption coefficient of the absorption lines of carbon dioxide and water molecules near 1.65 µm is much smaller than that of methane molecules[
A laser in the 1.6 µm band is designed as a potential light source for methane gas detection. It is required that the laser operates single mode lasing without mode hopping in the wavelength tuning range covering the methane absorption line at 1653.72 nm. The laser structure of a square microcavity as a WGM microcavity coupled with a Fabry–Pérot (FP) cavity meets the requirements and has the advantages of narrow linewidth, high side mode suppression ratio (SMSR), stable output wavelength, low power consumption, small volume, simple structure, and so on[
In this Letter, we report a square-FP coupled cavity semiconductor laser operating at about 1654 nm, corresponding to the methane absorption line. The laser output optical power can reach 7.4 mW with the SMSR about 40 dB, and the linewidth is 5 MHz at 1653.72 nm. The wavelength tunable properties of the coupled cavity laser were analyzed. The lasing wavelength can be tuned by adjusting the FP cavity injection current covering a 2 nm tuning range, and it can also be tuned by adjusting the square microcavity injection current or temperature, respectively. A gas detection system based on TDLAS technology using the coupled cavity laser was demonstrated and used for methane gas detection.
2. Device Structure Simulation and Fabrication
We numerically simulate the field distributions of the magnetic component in hertz (Hz) for transverse electric (TE) polarized modes of a 2D square-FP coupled cavity with an FP cavity length of 300 µm and width of 2.5 µm as well as a square microcavity side length of 15 µm by the finite element method in the commercial software COMSOL Multiphysics 5.0. The coupled cavity with refractive index
Figure 1.Square-FP coupled cavity 2D structure schematic.
Figure 2.TE mode distributions of the coupled cavity at different wavelengths. (a) 1642.8 nm; (b) 1641.47 nm; (c) 1641.0 nm.
An AlGaInAs/InP multiple-quantum-well laser wafer is used to fabricate the coupled cavity laser. The laser adopts the structure of numerical simulation above, as shown in Fig. 1. First, a 600 nm
Figure 3.Microscope image of the square-FP coupled cavity laser (a) after cleaving and (b) after wedge bonding.
3. Device Characteristics
The coupled cavity laser is tested on a semiconductor thermoelectric cooler (TEC), which is fixed at 16°C except for the variable temperature test. A multi-mode fiber is used to collect the output light at the output facet of the FP cavity away from the square microcavity except for the linewidth characterization. The injection currents of the two
Figure 4.Output optical power with the variation of IFP at ISQ = 20 mA. Inset: the output optical power with the variation of ISQ at IFP = 83 mA.
Figure 5.Optical spectrum of the laser at injection current of ISQ = 20 mA and IFP = 84 mA.
The injection currents of the two
Figure 6.(a) Optical spectra and SMSR with the variation of IFP at ISQ = 20 mA; (b) spectra with the variation of ISQ at IFP = 83 mA.
The lasing wavelength can also be tuned by adjusting the temperature except for adjusting the injection current, and it is redshifted with increasing temperature. This is because the energy band structure and Fermi distribution in semiconductors are temperature dependent, and the active region gain peak and material refractive index change with temperature. For the coupled cavity laser, the lasing wavelength shift with temperature depends on material refractive index variation, rather than the gain spectrum shift. Optical spectra and SMSR of the laser with temperature variation of the TEC at
Figure 7.Optical spectra and SMSR of the laser with the variation of temperature from 4.3°C to 37.7°C.
The linewidth of the coupled cavity laser is characterized at
Figure 8.Linewidth of the laser at injection current of ISQ = 20 mA and IFP = 84 mA.
4. Methane Gas Detection
A gas detection system based on TDLAS technology using the coupled cavity laser proposed in this Letter was built for methane gas detection. A white cell is used as the gas absorption cell. The sample gas is methane with a concentration of 1001 ppm (parts per million), and its equilibrium gas is nitrogen. The gas cell is filled with air before the sample gas is inlet. The lasing wavelength is tuned from 1653.65 to 1653.8 nm by adjusting
Figure 9.(Pair-PCH4)/Pair with the variation of the lasing wavelength.
5. Conclusion
In this Letter, methane gas detection is successfully demonstrated utilizing a square-FP coupled cavity laser. The coupled cavity laser operates at about 1654 nm, corresponding to the methane absorption line. The laser can operate single mode lasing with the SMSR about 40 dB and the linewidth of 5 MHz, and optical power can reach 7.4 mW. The lasing wavelength can be tuned by adjusting
References
[1] D. F. Swineharf. The Beer–Lambert law. J. Chem. Educ., 39, 333(1962).
[2] P. Werle. A review of recent advances in semiconductor laser based gas monitors. Spectrochim. Acta A Mol. Biomol. Spectrosc., 54, 197(1998).
[3] P. Werle, F. Slemr, K. Maurer, R. Kormann, R. Mucke, B. Janker. Near- and mid-infrared laser-optical sensors for gas analysis. Opt. Laser. Eng., 37, 101(2002).
[4] N. S. Lawrence. Analytical detection methodologies for methane and related hydrocarbons. Talanta, 69, 385(2006).
[5] M. Lackner. Tunable diode laser absorption spectroscopy (TDLAS) in the process: a review. Rev. Chem. Eng., 23, 65(2007).
[6] J. Shemshad, S. M. Aminossadati, M. S. Kizil. A review of developments in near infrared methane detection based on tunable diode laser. Sensor. Actuat. B Chem., 171, 77(2012).
[7] J. Kamieniak, E. P. Randviir, C. E. Banks. The latest developments in the analytical sensing of methane. TrAC Trend. Anal. Chem., 73, 146(2015).
[8] S. V. Kireev, S. L. Shnyrev. On-line monitoring of odorant in natural gas mixtures of different composition by the infrared absorption spectroscopy method. Laser Phys. Lett., 15, 035705(2018).
[9] F. Wang, S. Jia, Y. Wang, Z. Tang. Recent developments in modulation spectroscopy for methane detection based on tunable diode laser. Appl. Sci., 9, 2816(2019).
[10] J. Mi, H. Yu, L. Yuan, S. Li, M. Li, S. Liang, Q. Kan, J. Pan. Distributed Bragg reflector laser (1.8 µm) with 10 nm wavelength tuning range. Chin. Opt. Lett., 13, 041401(2015).
[11] H. Yu, P. Wang, J. Mi, X. Zhou, J. Pan, H. Wang, L. Xie, W. Wang. 1.8-µm DBR lasers with over 11-nm continuous wavelength tuning range for multi-species gas detection. Asia Communications and Photonics Conference (ACP), 1(2017).
[12] H. Yu, J. Pan, X. Zhou, H. Wang, L. Xie, W. Wang. A widely tunable three-section DBR lasers for multi-species gas detection. Appl. Sci., 11, 2618(2021).
[13] H. Yu, M. Wang, D. Zhou, X. Zhou, P. Wang, S. Liang, Y. Zhang, J. Pan, W. Wang. A 1.6-µm widely tunable distributed Bragg reflector laser diode based on InGaAs/InGaAsP quantum-wells material. Opt. Commun., 497, 127201(2021).
[14] Y. Fu, H. Liu, Y. Sui, B. Li, W. Ye, C. Zheng, Y. Wang. A near-infrared methane detection system using a 1.654 µm wavelength-modulated diode laser. Optoelectron. Lett., 12, 140(2016).
[15] J. Wang, B. Li, G. Lin, Q. Ma, S. Wang, M. Piao. Near-infrared methane sensor based on a distributed feedback laser. Spectrosc. Lett., 52, 113(2019).
[16] L. Shao, B. Fang, F. Zheng, X. Qiu, Q. He, J. Wei, C. Li, W. Zhao. Simultaneous detection of atmospheric CO and CH4 based on TDLAS using a single 2.3 µm DFB laser. Spectrochim. Acta A Mol. Biomol. Spectrosc., 222, 117118(2019).
[17] B. Wang, H. Lu, A. Li, Y. Chen, T. Dai, S. Huang, H. Lian. Research of TDLAS methane detection system using VCSEL laser as the light source. Infrared Laser Eng., 49, 0405002(2020).
[18] M. Chen, Y. Shi, R. Xiao, Z. Sun, S. Chen, Y. Xu, B. Yang, X. Chen. Tunable DFB laser array for multi-gas detection. 19th International Conference on Optical Communications and Networks (ICOCN), 1(2021).
[19] B. Li, L. Xue, N. Ji, D. Wei. Research on spectroscopy modulation of a distributed feedback laser diode based on the TDLAS technique. Int. J. Opt., 2021, 8829790(2021).
[20] F. Meng, H. Yu, X. Zhou, Y. Li, M. Wang, W. Yang, W. Chen, Y. Zhang, J. Pan. Quantum wells micro-ring resonator laser emitting at 1746 nm for gas sensing. Chin. Opt. Lett., 19, 041406(2021).
[21] H. Lian, B. Wang, Y. Yu, L. Cheng, T. Dai, S. Huang. Carbon monoxide gas detection system based on VCSEL using TDLAS technology. Proc. SPIE, 11887, 118871O(2021).
[22] I. E. Gordon, L. S. Rothman, C. Hill, R. V. Kochanov, Y. Tan, P. F. Bernath, M. Birk, V. Boudon, A. Campargue, K. V. Chance, B. J. Drouin, J. M. Flaud, R. R. Gamache, J. T. Hodges, D. Jacquemart, V. I. Perevalov, A. Perrin, K. P. Shine, M. A. H. Smith, J. Tennyson, G. C. Toon, H. Tran, V. G. Tyuterev, A. Barbe, A. G. Csaszar, V. M. Devi, T. Furtenbacher, J. J. Harrison, J. M. Hartmann, A. Jolly, T. J. Johnson, T. Karman, I. Kleiner, A. A. Kyuberis, J. Loos, O. M. Lyulin, S. T. Massie, S. N. Mikhailenko, N. Moazzen-Ahmadi, H. S. P. Muller, O. V. Naumenko, A. V. Nikitin, O. L. Polyansky, M. Rey, M. Rotger, S. W. Sharpe, K. Sung, E. Starikova, S. A. Tashkun, J. Vander Auwera, G. Wagner, J. Wilzewski, P. Wcislo, S. Yu, E. J. Zak. The HITRAN2016 molecular spectroscopic database. J. Quant. Spectrosc. Radiat. Transfer, 203, 3(2017).
[23] T. Hosoda, G. Kipshidze, L. Shterengas, G. Belenky. Diode lasers emitting near 3.44 µm in continuous-wave regime at 300 K. Electron. Lett., 46, 1455(2010).
[24] L. Naehle, S. Belahsene, M. von Edlinger, M. Fischer, G. Boissier, P. Grech, G. Narcy, A. Vicet, Y. Rouillard, J. Koeth, L. Worschech. Continuous-wave operation of type-I quantum well DFB laser diodes emitting in 3.4 µm wavelength range around room. Electron. Lett., 47, 46(2011).
[25] G. K. Veerabathrana, S. Sprengel, A. Andrejew, M.-C. Amann. Room-temperature vertical-cavity surface-emitting lasers at 4 µm with GaSb-based type-II quantum wells. Appl. Phys. Lett., 110, 071104(2017).
[26] H. Nie, F. Wang, J. Liu, K. Yang, B. Zhang, J. He. Rare-earth ions-doped mid-infrared (2.7–3 µm) bulk lasers: a review. Chin. Opt. Lett., 19, 091407(2021).
[27] X. Ma, Y. Huang, Y. Yang, J. Xiao, H. Weng, Z. Xiao. Mode coupling in hybrid square-rectangular lasers for single mode operation. Appl. Phys. Lett., 109, 071102(2016).
[28] X. Ma, Y. Huang, Y. Yang, H. Weng, J. Xiao, M. Tang, Y. Du. Mode and lasing characteristics for hybrid square-rectangular lasers. IEEE J. Sel. Top. Quantum Electron., 23, 1500409(2017).
[29] Y. Hao, F. Wang, M. Tang, H. Weng, Y. Yang, J. Xiao, Y. Huang. Widely tunable single-mode lasers based on a hybrid square/rhombus-rectangular microcavity. Photonics Res., 7, 543(2019).
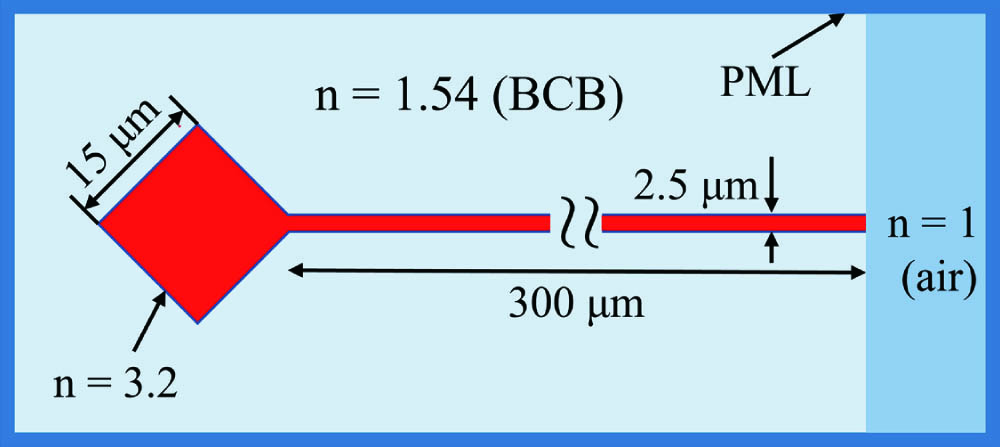
Set citation alerts for the article
Please enter your email address