Author Affiliations
1Zhejiang University, College of Optical Science and Engineering, State Key Laboratory of Extreme Photonics and Instrumentation, Hangzhou, China2The Chinese University of Hong Kong, Department of Biomedical Engineering, Hong Kong, China3China Jiliang University, College of Optical and Electronic Technology, Hangzhou, China4Zhejiang University of Technology, Institute of Pharmacology, College of Pharmaceutical Sciences, Hangzhou, China5ZJU-Hangzhou Global Scientific and Technological Innovation Center, Hangzhou, China6Huazhong University of Science and Technology, Britton Chance Center for Biomedical Photonics-MoE Key Laboratory for Biomedical Photonics, Advanced Biomedical Imaging Facility-Wuhan National Laboratory for Optoelectronics, Wuhan, China7Shanxi University, Collaborative Innovation Center of Extreme Optics, Taiyuan, Chinashow less
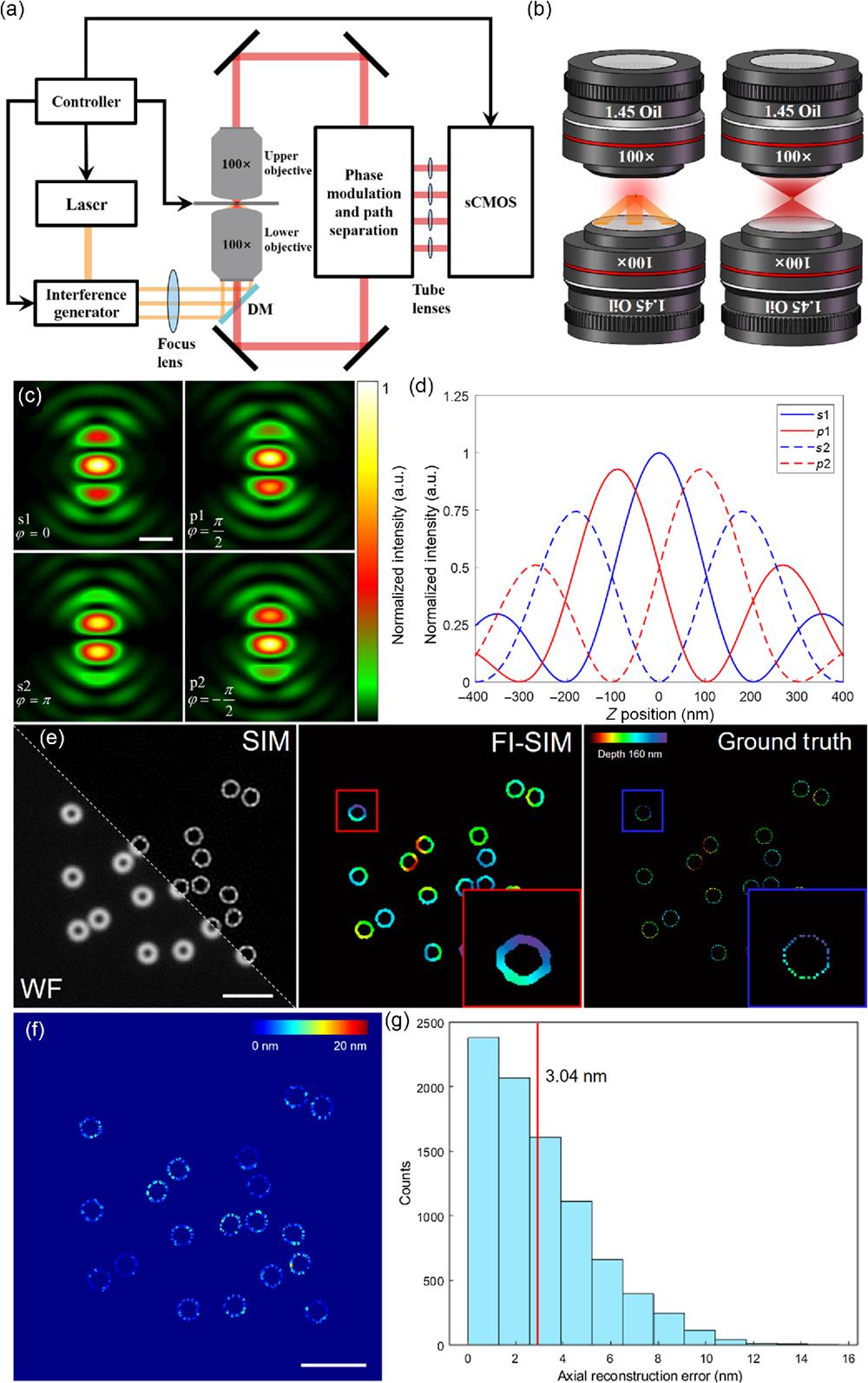
Fig. 1. Principle and simulations of FI-SIM. (a) Structure schematic diagram of FI-SIM. Excitation beam was split by the interference generator module, which contains galvo-mirrors and piezo stages to form a three-beam illumination mode (3D-SIM); fluorescence from both upper and lower objectives would interfere and be modulated by phase modulation and path separation modules to form four paths and correspondingly four sub-images, which denoted four different phases of fluorescence interference; the images were collected by sCMOS, and all of the devices were synchronously controlled by computer. (b) Excitation and detection mode of FI-SIM. (Left) For excitation, only the lower objective is used, and the pattern is generated by three-beam illumination, which is the same as 3D-SIM but without axial scanning. (Right) For detection, both objectives collect the fluorescence from the sample, resulting in fluorescence interference. (c) 4Pi PSF of four sub-images with four phases (, 0, , ) theoretically. (d) Signal intensities of four interference images as a function of axial positions. (e) Simulation of ring-shaped structures. (Left) Wide-field (WF) image and SIM reconstructed image; (middle) axial reconstruction after spatial mask of SIM results; (right) ground truth. Expansion of the red and blue outlined region of the image in (e) denotes the image details of corresponding region in FI-SIM and ground truth, respectively. (f) Error map between FI-SIM and ground truth. (g) Frequency histogram of axial reconstruction error after pixel-by-pixel axial reconstruction by FI-SIM. Scale bar in (c) 400 nm; in (e) and (f) .
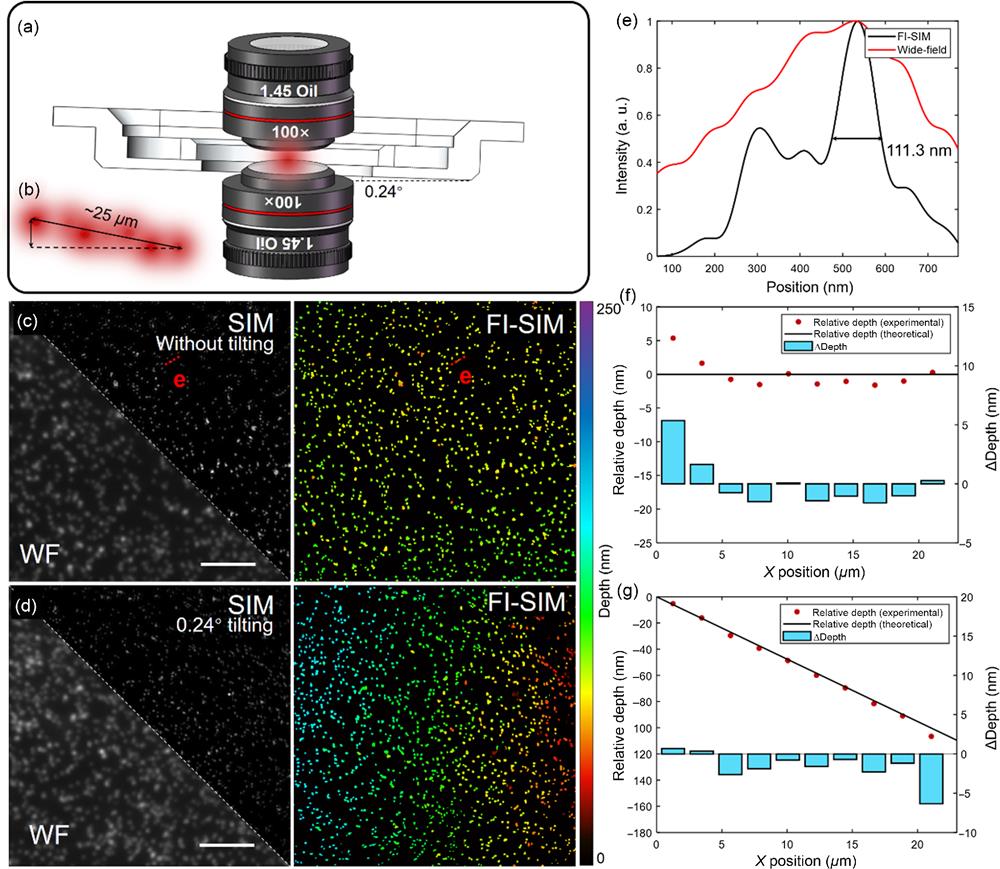
Fig. 2. Calibration by fluorescent beads and schematic diagram of sample stage tilting. (a) Schematic diagram of 0.24 deg tilting between the sample stage and axis, which generates difference in axial direction from leftmost to rightmost of the ROI. (b) Expansion of the red outlined region in (a), the size of the FOV is , which is consistent with images in (c) and (d). (c) WF, SIM, and axial reconstruction result when sample stage stayed level. (Left) WF and SIM image; (right) 3D volume super-resolution image. (d) WF, SIM, and axial reconstruction result when sample stage tilts 0.24 deg. (Left) WF and SIM image; (right) 3D volume super-resolution image. (e) Lateral profile of the red line in (c) and the FWHM is 111.3 nm. (f) In (c), regard the average axial position of the leftmost fluorescent beads in the FOV as 0 nm and measure the average relative depth (axial position) of the fluorescent beads every along the direction in the FOV. The red points represent the average values of the relative depth at corresponding -coordinate, the black line represents the theoretical relative depths along the direction, and the blue histogram represents the differences between the actual relative depths and corresponding theoretical values. (g) In (d), regard the average axial position of the leftmost fluorescent beads in the FOV as 0 nm and measure the average relative depth (axial position) of the fluorescent beads every along the direction in the FOV. The red points represent the average values of the relative depth at corresponding coordinate, the black line represents the theoretical relative depths along the direction, and the blue histogram represents the differences between the actual relative depths and corresponding theoretical values. Scale bar: .
Fig. 3. Reconstructed image and 3D volume of microtubules and migrasomes. (a) 3D-SIM image without axial scanning. The top-left of (a) is the corresponding wide-field image. (b) 3D volume super-resolution image of microtubules labeled with STAR Orange in BSC cells. (c) Lateral profile of the white line in (b) and the FWHM is 185.6 nm. (d) 3D visualization of the microtubules using Vutara SRX Viewer. (e) Continuous – section of the 3D volume from 0 to 66 nm depth with a 5-nm axial interval, illustrating the changing morphology of microtubule networks in a narrow axial range. (f) 3D-SIM image without axial scanning. (g) 3D volume super-resolution image of migrasomes labled with DID in live L929 cells. (h) Lateral profile of the white line in (g). (i) 3D volume visualization of the microtubules using Vutara SRX Viewer. (j) Continuous – section of the 3D volume from 0 to 220 nm depth with a 20-nm axial interval. Scale bar: .
Fig. 4. Reconstructed results of live-cell time-lapse imaging of microtubules and mitochondria. (a), (f) Wide-field image of microtubules and mitochondria in live U2OS cells labeled with Tubulin-Tracker Deep Red and Mito-Tracker Deep Red, respectively. (b), (g) 3D-SIM image of microtubules and mitochondria. (c), (h) Time-lapse wide-field image sequence of the ROIs marked with red outline in (a) and (f) during 2 min with time interval of one minute. (d), (i) Time-lapse 3D-SIM image sequence of the ROIs marked with the red outlined region in (b) and (g). (e), (j) Time-lapse depth-coded 3D volume image sequence of the ROIs marked as red outlined regions in (b) and (g). Scale bar in (a) and (f): ; scale bar in (c) and (h): .
Fig. 5. Reconstructed results of live-cell fast image acquisition of migrasomes in live L929 cells labeled with DID. (a) 3D-SIM image of migrasomes in live U2OS cells labeled with Tubulin-Tracker Deep Red. (b) Wide-field image of migrasomes. (c), (d), and (e) Time-lapse FI-SIM morphology image sequence of the different ROIs marked with red outline in (a) with fast image acquisition. Scale bar in (a) and (b): ; scale bar in (c), (d), and (e): .