
- Chinese Optics Letters
- Vol. 19, Issue 8, 081405 (2021)
Abstract
Keywords
1. Introduction
The development of ultrafast laser technology has become one of the most cutting-edge domains and hot points in photonics and even modern science. Ultrafast laser technology is expected to make a breakthrough in numbers of fields and plays an important role in promoting the future development of science and technology. As one of the greatest inventions in the 20th century, the development of lasers has gone through nearly 60 years since the first, to the best of our knowledge, demonstration of coherent light generated from a flashlamp-pumped ruby crystal[
Mode-locking, which usually incorporates either active or passive pulse modulators, is a commonly used technique to generate ultrashort pulses. More specifically, active mode-locking requires an external signal normally driven by an acousto-optic or electro-optic modulator. A typical external sinusoidal signal periodically modulates the intracavity loss, the modulation rate of which is integer multiples of the cavity frequency[
Here, we review the recently studied advances of SAs in terms of fabrication and applications. In Section 2, we mainly introduced the fundamental properties of the most widely studied semiconductor materials including SESAMs, carbon nanotubes (CNTs) and graphene, and transition metal dichalcogenides (TMDCs). This was then followed by the fabrication of the SAs and their ultrafast laser applications both in free-space and fiber laser systems. In the last section, we discussed the pros and cons of the present SA fabrication methods and ended up with perspectives on LDMs-based SAs.
Sign up for Chinese Optics Letters TOC. Get the latest issue of Chinese Optics Letters delivered right to you!Sign up now
2. Fundamental of Properties of Saturable Absorbers
Saturable absorption properties are of great significance for passively mode-locked lasers. Pulse operation initiates from the random noise sequence in the laser cavity. When an optical pulse propagates through the SA, its wings experience more loss than the central part, because high intensity more easily saturates the absorber. As a result, the loss modulation is synchronized automatically with the laser pulses. The Pauli blocking effect can explain the saturable absorption phenomenon at the micro-level. When high-intensity light interacts with a nonlinear optical material, electrons in the valence band absorb the incident photons and transit to a higher conduction band. For low-incident intensity, most of the photons are absorbed, hence, resulting in low transmission. Under high incident beam intensity, the conduction band is full with electrons and cannot accept more electrons due to the Pauli blocking effect. Consequently, most of the photons transit through the material resulting in high transmission. The basic mechanism for mode-locking can be easily understood by considering the SA as a passive optical modulator whose absorption (loss) is intensity-dependent, which can be described by a two-level model:
2.1. SESAMs
Since 1990, Keller et al. have attempted to implement semiconductor SAs into lasers for self-starting mode-locking[
For practical purposes, we assume that
Besides, saturation fluence plays an important role in the reduction of the mode-locking threshold and the avoidance of optical damage. The saturation energy of commercial SESAMs is typically centered between
2.2. Low-dimensional materials
2.2.1. CNTs and graphene
The unique lattice structure of carbon-based LDMs (i.e., CNTs, graphene) endows them with superior linear and nonlinear optical properties. The explosion in research of CNTs was initiated by the group of Iijima in 1991[
Graphene is a semimetal with a honeycomb lattice structure. The linear absorption of single-layer graphene is determined by the fine structure constant (
2.2.2. TMDCs
Similar with graphene, bulk TMDCs are formed by stacking single-material layers with weak van der Waals forces. TMDCs exhibit rich physical behaviors from wideband insulators to narrowband semiconductors, semimetals, or metals, which offer evident optical responses over an extremely wide spectral range (from ultraviolet to terahertz or even microwave)[
3. Fabrication and Ultrafast Laser Applications of SAs
3.1. SESAMs mode-locked lasers
The emergence of SESAMs is considered the revolutionary improvement for ultrafast lasers. Molecular beam epitaxy (MBE) and metal-organic vapor phase epitaxy (MOVPE) are two generally used fabrication techniques with different growth pressures and temperatures. A SESAM typically consists of a high-quality distributed Bragg reflector (DBR) followed by a multi-QWs or QDs absorption layer. The design of the DBR and absorption region has been optimized for desirable optical parameters, such as the modulation depth, saturation energy, non-saturable losses, and recovery time[
After nearly a decade of efforts, SESAM mode-locking has motivated the frontier in hundred GHz repetition rates (up to more than 160 GHz at 1 µm and 100 GHz at 1.5 µm)[
Figure 1.SESAM-based mode-locked Yb:YAG TDL. (a) Schematic of the cavity design. (b) Beam profile at 350 W output power in CW operation. (c) Beam profile at 350 W in mode-locked operation. The vertical and lateral cuts of the beam are depicted as red lines with Gaussian fits. (d) Optical spectrum with the central wavelength of 1030 nm. (e) Autocorrelation (AC) trace. (f) Radio frequency (RF) spectrum with 42 dB signal-to-noise ratio. (a)–(f) Reproduced with permission[
SESAM mode-locked solid-state lasers have stimulated the novel ultrafast semiconductor disk laser (SDL), which bridges the gap between the solid-state laser and the solid-state TDL. VECSELs combining the advantage of semiconductor gain materials and SESAMs possess large gain cross section and are therefore ideally suited for high repetition rates, high average output powers generation. Additionally, advantages such as mass production on the wafer scale and easy integration into complex optical circuits are important for practical applications[
Figure 2.Quantum dots (QDs) as active media for ultrafast mode-locked VECSEL. (a) V-shaped cavity designed with the SESAM and output coupler as end mirrors and the VECSEL gain chip as the folding mirror. (b) Epitaxial structure of the QD VECSEL and the standing wave intensity profile at the central wavelength of 1035 nm. (c) and (d) SESAM mode-locked VECSEL results with different cavity designs. (c) Pulse characterizations are measured with a pulse duration of 216 fs and a full width at half-maximum (FWHM) of 5.7 nm at 2.77 GHz repetition rate. (d) Pulses of 193 fs with 6.6 nm FWHM bandwidth and 1.67 GHz repetition rate. Reproduced with permission[
Many applications require more compact and simpler fs sources with a minimum number of components. A logical following step toward more compact SDL is the MIXSEL, which integrates both the gain and the absorber within one wafer to improve the integration level so that stable self-starting mode-locking can be achieved in a simple straight cavity. After the first, to the best of our knowledge, demonstration of optically pumped MIXSEL in 2007[
Figure 3.Optically pumped high-power MIXSEL. (a) MIXSEL concept and SEM image: the MIXSEL semiconductor consists of two highly distributed Bragg reflectors (DBRs), a QD saturable absorption layer, a quantum well (QW) gain section, and an anti-reflective (AR) coating. (b) Photograph and sketch of the MIXSEL cavity: the sample straight cavity is formed by a MIXSEL chip and an output coupler. (c) RF spectrum, (d) optical spectrum, (e) AC trace intensity, and (f) beam quality measurement. (a)–(f) Reproduced with permission[
The wavelength flexibility and controllable fabrication make SESAMs superior to other types of SAs. However, limited by the available gain materials and saturable absorption materials, SESAMs mode-locking in the MIR or extreme ultraviolet (XUV) have not reached the same mature degree as in the NIR. In 2017, Labaye et al. reported the first intracavity high-harmonic generation (HHG) inside a TDL oscillator for XUV light generation[
Figure 4.XUV light source generation based on HHG inside a mode-locked TDL. (a) Illustration of the experimental setup. (b) AC trace and (c) RF spectrum without a high-pressure xenon gas jet. (d) AC trace and (e) RF spectrum with high-pressure xenon gas jet. (f) Optical spectrum of the generated XUV light. (g) Amplitude and phase noise measurements of the mode-locked TDL with and without gas. (a)–(g) Reproduced with permission[
Figure 5.SESAM mode-locked
More details are listed in Table 1, which collects some typical laser performances of SESAM mode-locking from visible to MIR wavelengths.
Gain materials | Modulation depth (%) | τp (fs)(a) | λ (nm)(b) | frep (MHz)(c) | Pout (mW)(d) | Slope efficiency (%) | Pump source(e) | Ref. |
---|---|---|---|---|---|---|---|---|
Pr3+:LiYF4 | \ | \ | 523 | \ | 2900 | 79 | 497 DL | [ |
Ti:sapphire | \ | 68 | 816 | 379 | 200 | 10 | InGaN DL | [ |
Yb:CNGG | 0.9 | 55 | 1051.5 | 87 | 60 | 1 | DBR DL | [ |
Yb,Na:CNGG | 0.6 | 45 | 1061 | 104 | 734 | 68.5 | 980 DL | [ |
Yb:CaGdAlO4 | \ | 166 | 1050 | 10.6 | 1200 | 2.8 | 980 DL | [ |
Yb:KGW | 0.5 | 56 | 1040 | 77.3 | 1950 | \ | 980 DL | [ |
Er:Yb:glass | 0.4 | 4700 | 1535 | 9788 | 9 | \ | 980 DL | [ |
Er:Yb:glass | \ | 5400 | 1544.4 | 6803.3 | 30 | \ | 980 DL | [ |
Tm,Ho:CaYAlO4 | \ | 87 | 2042.6 | 80.45 | 27 | 22 | TSL | [ |
Tm:LuScO3 | 1 | 170 | 1973–2142 | 115.2 | 190 | 33 | TSL | [ |
Cr:ZnSe | \ | 408 | 2042 | 127 | 403 | 12.2 | TDFL | [ |
Tm:(Lu2/3Sc1/3)2O3 ceramic | \ | 74 | 2057 | 78.9 | 175 | 34 | TSL | [ |
Tm,Ho:CNGG | \ | 73 | 2061 | 89.3 | 36 | \ | TSL | [ |
Cr:ZnSe | \ | 100 | 2450 | 215 | 100 | \ | EDFL | [ |
Cr:ZnS | \ | 130 | 2375 | 180 | 130 | \ | EDFL | [ |
Yb:Lu2O3 disk | 1.1 | 616 | 1033 | 10 | 82,000 | 44 | DL | [ |
Yb:YAG disk | 2.7 | 780 | 1030 | 10.96 | 210,000 | \ | 940 DL | [ |
Yb:YAG disk | 1.1 | 940 | 1030 | 8.88 | 350,000 | \ | DL | [ |
Yb:Lu2O3 disk | 1.6 | 255 | 60.8 | 17.35 | 320,000 | \ | 976 DL | [ |
VECSEL | 1.4 | 216 | 1035 | 2770 | 269 | 2.1 | DL | [ |
VECSEL | \ | 95 | 1025 | 2200 | 90 | \ | 790 DL | [ |
VECSEL | 0.5 | 682 | 1030 | 1710 | 5100 | \ | 808 DL | [ |
VECSEL | \ | 400 | 1013 | 1670 | 3300 | 2.9 | 808 DL | [ |
VECSEL | 2 | 5000 | 1341 | 1030 | 1670 | \ | 980 DL | [ |
MIXSEL | \ | 570 | 964 | 101.2 | 127 | \ | 808 DL | [ |
MIXSEL | \ | 28,100 | 959 | 2470 | 6400 | 17.3 | 808 DL | [ |
MIXSEL | \ | 144 | 1033 | 2730 | 30 | <0.5 | 808 DL | [ |
Table 1. Passively Mode-Locked Solid-State Lasers Based on SESAMs.
3.2. LDMs-based mode-locked lasers
3.2.1. LDMs-SAs for solid-state lasers
The fabrication of LDMs-based SAs is usually directly depositing or transferring nanomaterials onto optical substrates to form reflection or transmission type structures. It is worth noting that the insertion loss should be well designed due to a much lower single-pass gain in solid-state lasers. Passively mode-locked all-solid-state lasers based on CNT-SAs have been successfully investigated from 0.8 to 2.1 µm wavelengths[
Figure 6.(a) Schematic of the mode-locked VECSEL cavity. OC, optical coupler mirror; HR, highly reflective folding mirror; GSAM, graphene SAM. (b) Picture of the GSAM by transferring single-layer graphene on an 8/λ
More details are listed in Table 2, which collects some typical laser performances of LDMs mode-locking in solid-state lasers.
Gain materials | Nanomaterials | Modulation depth (%) | τp (ps) | λ (nm) | frep (MHz) | Pout (mW) | Pump source | Ref. |
---|---|---|---|---|---|---|---|---|
Ti:sapphire | SWCNT | \ | 0.2 | 810 | 110 | 45 | AL | [ |
Ti:sapphire | SWCNT | 0.15 | 0.062 | 800 | 99.4 | 600 | 532 nm DL | [ |
Yb:KLuW | SWCNT | 0.25 | 0.115 | 1045 | 89 | 53 | TSL | [ |
Yb:KYW/KYW | SWCNT | 0.21 | 0.083 | 1038 | 84 | 24 | TSL | [ |
Yb:YAG | SWCNT | \ | 2 | 1030.3 | 2080 | 322 | DL | [ |
Cr:forsterite | SWCNT | 0.4 | 0.12 | 1250 | 79.1 | 202 | YFL | [ |
Tm:CNNGG | SWCNT | 0.5 | 0.084 | 2018 | 89.9 | 22 | TSL | [ |
Cr:ZnS | CNT | \ | 0.061 | 2350 | 250 | 950 | EDFL | [ |
Tm:KY(WO4)2 | PbS QD | \ | \ | 1936 | 185 | 20 | 802 DL | [ |
Yb:YOCB | Graphene | \ | 0.152 | 1037 | 99.9 | 53 | 980 DL | [ |
Tm:CLNGG | Graphene | \ | 0.354 | 2010 | 98 | 97 | 790 DL | [ |
Cr:ZnSe | Graphene | \ | 0.116 | 2352 | 99 | 66 | EYFL | [ |
Nd:YAG | Graphene | \ | 16 | 1064 | 11,500 | 12 | 809 DL | [ |
CrZnS | Graphene | \ | 0.041 | 2370 | 108 | 250 | EDFL | [ |
Nd:YVO4 | MoS2 | 7 | 12.7 | 1064.2 | 88.3 | 89 | 808 DL | [ |
Nd:LuVO4 | PtSe2 | 12.6 | 15.8 | 1066.573 | 61.3 | 180 | 808 DL | [ |
VECSEL | SWCNT | 0.25 | 1.23 | 1074 | 613 | 136 | DL | [ |
VECSEL | Graphene | 0.55 | 0.466 | 949 | 2480 | 26 | 808 DL | [ |
Table 2. Passively Mode-locked Solid-state Lasers Based on LDM-SAs
3.2.2. LDMs-SAs for fiber lasers
Recently, the study on fiber lasers has shown explosive growth owing to their inherent advantages in the respect of flexibility, high beam quality, alignment-free format, and favorable heat sinking. LDMs can be easily integrated into various optical configurations to achieve alignment-free and all-fiber formats, which cannot be realized for SESAMs.
A. LPE technique and ink-jet printing method
The solution-processing technique is widely used for high-yield few-layer nanosheets preparation, which includes either chemical exfoliation (e.g., lithium ion intercalation) or liquid phase exfoliation (LPE)[
Figure 7.SWCNT-PVA mode-locked fiber laser with stretched pulse generation. (a) Schematic of the laser setup and the dispersion distribution in the laser cavity. Normal dispersion is provided by the Er-doped fiber (EDF) and anomalous dispersion is provided by the Flexcore 1060 and SMF-28 fibers. WDM, wavelength division multiplexer; SMF, single-mode fiber; ISO, isolator; PC, polarization controller. (b) The nonlinear transmittance of SWCNT-PVA, which gives the modulation depth of 17%. (c) Optical spectrum with the bandwidth of 63 nm. (d) AC trace with a Gaussian fit. (a)–(d) Reproduced with permission[
Although excellent laser output performances have been obtained with fiber-end-face-integrated SAs, the mode-locking stability gradually deteriorates by increasing the pump power. Therefore, tapered-fiber (TF) and side-polished fiber (SPF)-based SA structures are proposed, in which the light–matter interaction is processed via the evanescent wave. The first, to the best of our knowledge, TF-integrated CNT mode-locked laser was reported in 2007, which delivered 694 fs/1.7 nJ pulses with a fundamental repetition rate at 13.3 MHz[
Figure 8.
Figure 9.Ultrafast laser mode-locking with double-covered
Although the LPE technique greatly facilitates the investigations on two-dimensional (2D) materials, the SA devices resulting from it suffer from the limitations of repeatability and durability. Inkjet printing provides a scalable craftsmanship that enables mask-less patterning with high resolution on flexible substrates. Indeed, 2D materials such as graphene,
Materials | Integration method(a) | λ (nm)(b) | SA properties(c) | Laser performances(d) | Ref. | |||||
---|---|---|---|---|---|---|---|---|---|---|
αs (%) | Is (MW·cm−2) | αns (%) | τ (fs) | frep (MHz) | P (mW) | SNR (dB) | ||||
SWCNT | Sandwiched | 1030 | 15 | \ | 44 | 235 | 50 | 155 | \ | [ |
SWCNT | Sandwiched | 1030 | 52.7 | 2.512 | 51 | 175 | 21.2 | 8.68 | 63 | [ |
SWCNT | Sandwiched | 1550 | 17 | 203 | \ | 74 | 33 | 1.2 | 76 | [ |
CNT | Sandwiched | 1928.5 | 13.3 | \ | \ | 501 | 56.368 | 28.5 | >70 | [ |
SWCNT | Sandwiched | 1560 | 16.9 | 18.9 | \ | 113 | 18.76 | 12.8 | 77 | [ |
SWCNT | SPF | 1563 | \ | \ | \ | 1020 | 38.9 | 250 | \ | [ |
CNT | FP mirror | 1563 | 5 | 2.5 | \ | 790 | 19,450 | \ | 27 | [ |
SWCNT | Sandwiched | 1927 | 10 | 68 | 14 | 152 | 25.76 | 4.85 | 73 | [ |
Graphene | Sandwiched | 1560 | 2 | 337 | 28 | 174 | 27.4 | \ | 87.4 | [ |
Graphene | Sandwiched | 1550 | \ | \ | \ | 263 | 18.67 | 0.68 | 63 | [ |
Graphene | Sandwiched | 1558 | 2 | 100 | 70 | 268 | 27.4 | 1.2 | 87.4 | [ |
Graphene | FP mirror | 1562 | \ | \ | \ | 865 | 9670 | \ | 40 | [ |
MoS2 | Sandwiched | 979 | 3.6 | 6.3 | 6.3 | 13,000 | 26.5 | 16.7 | 60 | [ |
MoS2 | TF | 1556.86 | 2.82 | \ | 57.34 | 3000 | 2500 | 5.39 | \ | [ |
MoS2 | Sandwiched | 1569.5 | 4.3 | 34 | 24 | 710 | 12.09 | 1.78 | 60 | [ |
WS2 | Sandwiched | 1572 | 2.9 | 370 | 30.9 | 919 | 25.3 | \ | 75 | [ |
WS2 | SPF | 1557 | 11 | 5 | 18 | 660 | 10.2 | \ | 65 | [ |
WS2 | TF | 1566 | 0.14 | \ | 82 | 467 | 21.1 | 0.32 | 60.8 | [ |
WS2 | TF | 1561 | 0.5 | \ | 21.4 | 369 | 24.93 | 1.93 | 69 | [ |
WSe2 | SPF | 1556.7 | 0.3 | \ | \ | 1310 | 3252.65 | 19 | \ | [ |
MoSe2 | Sandwiched | 1560 | 7.3 | \ | \ | 580 | 8.8 | 218 | 50 | [ |
ReS2 | Sandwiched | 1557 | 0.12 | 74 | \ | 1600 | 5.48 | 0.4 | \ | [ |
SnS2 | Sandwiched | 1562 | 4.6 | 125 | 13.6 | 623 | 29.33 | 1.2 | 45 | [ |
Bi2Se3 | Sandwiched | 1557 | 3.9 | 12 | 68 | 660 | 12.5 | 1.8 | 55 | [ |
Bi2Te3 | Sandwiched | 1557 | 2 | 180 | 34 | 1080 | 8.64 | 0.11 | 60 | [ |
Bi2Te3 | TF | 1560 | 1.82 | \ | 67 | 2180 | 3125 | \ | \ | [ |
Bi | TF | 1561 | 5.6 | 48.2 | 62.3 | 193 | 8.8 | 5.6 | 55 | [ |
BP | Sandwiched | 1560.5 | 4.6 | \ | 48 | 272 | 28.2 | 0.5 | 65 | [ |
BP | Sandwiched (printed) | 1555 | 10.03 | 14.98 | 9.97 | 102 | 23.9 | 1.7 | 60 | [ |
BP | Sandwiched (printed) | 1562 | \ | \ | \ | 605 | 31.6 | \ | 56 | [ |
TiS2 | Sandwiched | 1569.5 | 62 | 1210 | \ | 1040 | 5.34 | \ | 66 | [ |
GaTe | TF | 1563.17 | 42.3 | \ | 7.1 | 408 | 36.5 | 13.2 | 80 | [ |
GeTe | TF | 1931.51 | 8.3 | \ | 76.7 | 983 | 35 | 16.2 | 87.6 | [ |
HfS2 | TF | 1561.8 | 15.7 | 8 | 20.6 | 221.7 | 21.45 | 89.4 | 68 | [ |
HfSe2 | TF | 1561.4 | 5.8 | 163.2 | 45.6 | 297 | 18.09 | 48.5 | 80 | [ |
AuTe2Se4/3 | Sandwiched | 1557.53 | 65.58 | 0.089 | 18.83 | 147.7 | 69.93 | 21.4 | 91 | [ |
Ti3CN | SPF | 1557 | 1.7 | \ | \ | 660 | 15.4 | 0.05 | 60 | [ |
Table 3. Passively Mode-Locked Fiber Lasers Based on LPE-SAs.
Figure 10.Ultrafast mode-locked laser using inkjet-printed BP SA. (a) Photograph of formulated BP ink. (b) Droplet drying process without (left) and with (right) introducing recirculated Marangoni flow. (c) Optical photographs (left) and atomic force microscope (AFM) images (right) of the dried droplets. (d) Dark field optical micrographs of the printed tracks on
Figure 11.Ultrafast mode-locking with inkjet-printed
B. PVD technique
Physical vapor deposition (PVD) is a widely used vacuum deposition technique to produce nanometer-thick 2D material films. In a typical PVD process, bulk materials can be vaporized by several techniques, such as bombardment of energic ions [i.e., magnetron sputtering deposition (MSD)], ultraviolet laser ablation [i.e., pulsed laser deposition (PLD)], or heating in a furnace [i.e., thermal evaporation deposition (TED)]. PLD and MSD are the most commonly used transfer-free methods to grow nanomaterial films directly on the target substrates at relatively low working temperature. Those remarkable advantages have motived researchers to re-focus on PVD methods to fabricate the desired SAs on various substrates.
In 2014, our group firstly, to the best of our knowledge, fabricated a novel topological isolator (TI) film on the TF by the PLD technique[
Materials | Integration method(a) | λ (nm)(b) | SA Properties(c) | Laser Performances(d) | Ref. | |||||
---|---|---|---|---|---|---|---|---|---|---|
αs (%) | Is (MW·cm−2) | αns (%) | τ (fs) | frep (MHz) | P (mW) | SNR (dB) | ||||
Bi2Te3 | TF | 1562.4 | 6.2 | 28 | 20 | 320 | 2950 | 45.3 | 75 | [ |
Bi2Te3 | TF | 1930.07 | 38 | 3.3 | 31.2 | 1240 | 14.51 | 130 | 84 | [ |
Bi2Te3 | TF | 1542 | 7.42 | 175 | \ | 70 | 95.4 | 63 | 65 | [ |
Bi2Te3 | SPF | 1047.1 | 0.5-2.9 | \ | \ | 5900 | 19.28 | 4 | 71 | [ |
Bi2Te3 | SPF | 1558 | 5.3-13 | \ | \ | 167 | 25.38 | 5.34 | 68 | [ |
WTe2 | TF | 1915.5 | 31 | 7.6 | 34.3 | 1250 | 18.72 | 39.9 | 95 | [ |
MoTe2 | TF | 1559.57 | 25.5 | 9.6 | 19.1 | 229 | 26.6 | 57 | 93 | [ |
1934.85 | 22.1 | 12.3 | 21.3 | 1300 | 15.37 | 212 | 84 | [ | ||
α-In2Se3 | TF | 1565 | 4.5 | 7.3 | 21.9 | 276 | 40.9 | 83.2 | 90 | [ |
1932 | 6.9 | 10.6 | 28.8 | 1020 | 15.8 | 112.4 | 90 | [ | ||
WS2 | TF | 1559.7 | 1.2 | 25 | \ | 452 | 1040 | 11.3 | 48 | [ |
WS2 | SAM | 1549.98 | 4.48 | 138 | 2 | \ | 396 | 6.2 | 56.3 | [ |
WS2/MoS2/WS2 | SAM | 1562.66 | 16.99 | 6.23 | 36.97 | 296 | 36.46 | 25 | 90.3 | [ |
MoS2-Bi2Te3-MoS2 | SAM | 1554 | 64.17 | 151.176 | 35.83 | 286 | 36.4 | 20 | 73 | [ |
Cd3As2 | Sandwiched | 1968.5 | 3.5 | 12 | \ | 1360 | 23.2 | 4.9 | 70 | [ |
1560 | 5.1 | 67 | \ | 920 | 17.6 | 0.7 | 70 | [ |
Table 4. Passively Mode-Locked Fiber Lasers Based on PVD-SAs.
Figure 12.High-energy soliton pulse generation by magnetron sputtering deposition (MSD) grown
Figure 13.Self-starting mode-locking by fiber-integrated
Figure 14.
C. CVD technique
Chemical vapor deposition (CVD) is a versatile, scalable, and industry compatible technique for the controllable synthesis of atomic thin LDM films. Until now, a variety of LDMs including graphene, TMDs, and TIs have been synthesized in the lab[
Figure 15.Broadband mode-locked fiber laser by a large-area
Materials | Integration method(a) | λ (nm)(b) | SA properties(c) | Laser performances(d) | Ref. | |||||
---|---|---|---|---|---|---|---|---|---|---|
αs (%) | Is (MW·cm−2) | αns (%) | τ (fs) | frep (MHz) | P (mW) | SNR (dB) | ||||
Graphene | Sandwiched | 1545 | 11 | \ | ∼64 | 88 | 21.15 | 1.5 | 65 | [ |
Graphene | Sandwiched | 1884 | 0.4 | \ | \ | 1200 | 20.5 | 1.35 | ∼70 | [ |
Graphene | TF | 1550 | 12 | 40 | 27 | 970 | 8.57 | \ | 65 | [ |
MoS2 | Sandwiched | 1568.9 | 35.4 | 0.35 | 34.1 | 1280 | 8.288 | 5.1 | 62 | [ |
MoS2 | Sandwiched | 1569.6 | \ | \ | \ | 1420 | 216 | 6.81 | 36.1 | [ |
WS2 | Sandwiched | 1568.3 | 15.1 | 157.6 | 45.9 | 1490 | 0.487 | 62.5 | 71.8 | [ |
WS2 | TF | 1565 | 0.5 | \ | \ | 332 | 31.11 | \ | 81.6 | [ |
MoSe2 | TF | 1552 | 22.57 | 7.747 | 46.46 | 207 | 64.56 | \ | 85 | [ |
WSe2 | Sandwiched | 1562 | 52.38 | 1.399 | 45.51 | 185 | 58.8 | 30 | 95 | [ |
WSe2 | TF | 1863.96 | 1.83 | 3.7 | 87 | 1160 | 11.36 | 32.5 | 53 | [ |
WSe2 | TF | 1556.42 | 54.5 | 2.97 | ∼10 | 477 | 14.02 | \ | 80 | [ |
WSe2 | TF | 1886.22 | 1.83 | \ | ∼90 | 1180 | 11.36 | 32.5 | 80 | [ |
MoTe2 | TF | 1930.22 | 5.7 | 8.3 | 70 | 952 | 14.353 | 36.7 | 87.8 | [ |
PtSe2 | TF | 1563 | 4.9 | 340 | ∼70 | 1020 | 23.3 | \ | 61 | [ |
Bi2Se3 | Sandwiched | 1557.9 | 15 | 6.59 | \ | \ | 1.71 | 82.6 | 42 | [ |
Table 5. Passively Mode-locked Fiber Lasers Based on CVD-SAs
3.2.3. LDMs for MIR fiber lasers
MIR (2–10 µm) laser sources are of particular interests for various applications due to the unique fingerprint molecule spectra and transparent atmosphere windows in this region. Owing to the water vapor absorption in the atmosphere, which will induce considerable non-saturable loss, all-solid-state lasers around or after 2.8 µm have not been realized yet. Fiber lasers, hence, display their unique superiority for mode-locking in this region. Fluoride fiber lasers (FFLs) with various cavity structures, gain fibers, and SAs have been realized in the
4. Discussion
As far as the reported results are concerned, the performance of SESAMs and LDM-SAs has improved steadily. The fabrication of SAs is improving toward being controllable, low cost, easy integration as well as mass production. Meanwhile, the operating bands of SAs are developing toward MIR and far-infrared wavelengths. To ensure further advance of SAs, there are several basic requirements for SAs. (1) Self-starting ability: the SA is required to achieve mode-locking immediately after being inserted into a laser cavity without introducing perturbations. (2) Durability: in order to achieve the long-term mode-locking operation under high pump power, the as-fabricated SAs should possess a higher damage threshold as well as a lower non-saturable loss. (3) Ultrafast recovery time: SAs with ultrafast recovery time are more capable of achieving shorter pulse widths. (4) Low-cost and controllable fabrication are important factors for industry compatibility.
SESAMs with good customization have been widely employed in commercial lasers. In the past few decades, many researches have been done to improve the performance of SESAMs. The development of VECSEL and MIXSEL chips has greatly simplified the cavity structures, which allow for generating GHz repetition rates and several kilowatts peak power directly from a laser cavity. However, a retaining limitation of SESAMs is inflexible, which does not meet the characteristics of all-fiber integration. In addition, SESAMs have limited bandwidth (
LDMs with features like flexibility and ultrafast and broadband photoresponsivity are considered to meet these requirements. Figure 16 summarizes the mode-locked pulse width with different SAs in wide operating wavelength ranges. We use several colors to distinguish different SA fabricating technologies. LPE is a simple, contactless micromechanical exfoliation technique for high-yield nanosheets production. However, there are glaring problems such as poor fabrication controllability and repeatability and mode-locking performance degradation for a long-term operation, which should not be neglected. Notably, the inkjet printing method provides a new platform for the mass production of SA devices, and the long-term operation with high stability can be achieved by encapsulating SAs with a hexagonal boron nitride (h-BN) layer. Another advantage of inkjet printing is the controllable printing of desired graphic arrays on a myriad of substrates, which has already been applied in various photoelectronic devices (e.g., ultrafast lasers, photodetectors, and wearable electronic devices).
Figure 16.Wide-spectral mode-locking with different 2D materials.
PVD is a transfer-free method to grow nanomaterials directly on the target substrates at relatively low working temperatures. The deposited films generally have good crystallinity after annealing. For fiber-end-face-integrated SAMs, selecting materials with an appropriate modulation depth is critical for self-starting mode-locking. This compact SA device with a protective gold layer can be applied for stable high-frequency (∼GHz) mode-locking. CVD fabricated SAs possess a low damage threshold due to the atomic thickness and cannot work steadily for long periods of time without encapsulation. In addition, the additional transfer processes to the target substrate are prone to introducing undesired physical damages or defects, causing higher non-saturable losses. Polymer as an auxiliary is often added during the transfer process. Rapid and residue-free transfer of CVD synthesized films is still a challenge so far.
5. Outlook
5.1. New SA structures
Different atomically thin 2D materials can be readily stacked together by van der Waals forces to form a heterostructure, which offers a flexible and easy approach for bandgap engineering. In comparison with a single material, the heterostructure exhibits excellent nonlinear optical properties due to strong interlayer coupling[
Metasurfaces, artificial materials made of subwavelength elementary cells, have emerged as promising platforms for unexpected physical properties generation. Recently, Wang et al. demonstrated that plasmonic gold metasurfaces with excellent saturable absorption properties could behave as reliable SAs[
5.2. Actively controllable devices
Active manipulation of light in an optical fiber has gained research interest due to its compatibility with numerous optical systems. In 2015, Lee et al. demonstrated an electronically tunable all-fiber graphene device by fabricating graphene-based field effect transistor (FET) on the SPF[
5.3. SAs for MIR wavelength
During the past two decades, various SAs have been adopted for FFL mode-locking. However, the output pulse durations were several to tens of picoseconds with low average powers. Mode-locking in the fs range around 2.8 µm was achieved by nonlinear polarization rotation (NPR) in 2015[
In summary, the overall development of SAs tends to compactness, controllable fabrication, and scalability as well as active controllability. It is crucial to put emphasis on SAs that are fully compatible with fiber lasers, which play an increasingly important role in industry and human life. The fabrication of reliable and effective SAs will open up new directions for mass production of next-generation lasers.
References
[1] T. H. Maiman. Stimulated optical radiation in ruby. Nature, 187, 493(1960).
[2] A. Javan, W. R. Bennett, D. R. Herriott. Population inversion and continuous optical maser oscillation in a gas discharge containing a He-Ne mixture. Phys. Rev. Lett., 6, 106(1961).
[3] E. Snitzer. Optical maser action of Nd+3 in a barium crown glass. Phys. Rev. Lett., 7, 444(1961).
[4] R. N. Hall, G. E. Fenner, J. D. Kingsley, T. J. Soltys, R. O. Carlson. Coherent light emission from GaAs junctions. Phys. Rev. Lett., 9, 366(1962).
[5] W. Dietel, E. Döpel, D. Kühlke, B. Wilhelmi. Pulses in the femtosecond range from a cw dye ring laser in the colliding pulse mode-locking (CPM) regime with down-chirp. Opt. Commun., 43, 433(1982).
[6] U. Morgner, F. X. Kärtner, S. H. Cho, Y. Chen, H. A. Haus, J. G. Fujimoto, E. P. Ippen, V. Scheuer, G. Angelow, T. Tschudi. Sub-two-cycle pulses from a Kerr-lens mode-locked Ti:sapphire laser. Opt. Lett., 24, 411(1999).
[7] M. T. Asaki, C. P. Huang, D. Garvey, J. Zhou, H. C. Kapteyn, M. M. Murnane. Generation of 11-fs pulses from a self-mode-locked Ti: sapphire laser. Opt. Lett., 18, 977(1993).
[8] A. Stingl, M. Lenzner, C. Spielmann, F. Krausz, R. Szipöcs. Sub-10-fs mirror-dispersion-controlled Ti:sapphire laser. Opt. Lett., 20, 602(1995).
[9] P. Antoine, A. L’huillier, M. Lewenstein. Attosecond pulse trains using high-order harmonics. Phys. Rev. Lett., 77, 1234(1996).
[10] K. T. Kim, C. M. Kim, M. G. Baik, G. Umesh, C. H. Nam. Single sub 50 attosecond pulse generation from chirp-compensated harmonic radiation using material dispersion.. Phys. Rev. A, 69, 051805(2004).
[11] A. V. Korzhimanov, A. A. Gonoskov, E. A. Khazanov, A. M. Sergeev. Horizons of petawatt laser technology. Phys.-Uspekhi, 54, 9(2011).
[12] F. Lureau, G. Matras, S. Laux, C. Radier, O. Chalus, O. Casagrande, C. Derycke, S. Ricaud, P. Calvet, L. Boudjemaa, C. S. Boisson, D. Ursescu, I. Dancus. 10 petawatt laser system for extreme light physics, ATh1A.5(2019).
[13] A. H. Zewail. Laser femtochemistry. Science, 242, 1645(1988).
[14] H. Jung, R. Stoll, X. Guo, D. Fischer, H. X. Tang. Green, red, and IR frequency comb line generation from single IR pump in AlN microring resonator. Optica, 1, 396(2014).
[15] M. Suh, Q. Yang, K. Y. Yang, X. Yi, K. J. Vahala. Microresonator soliton dual-comb spectroscopy. Science, 354, 600(2016).
[16] J. Wu, Y. Xu, J. Xu, X. Wei, A. Chan, A. Tang, A. Lau, B. Chung, H. Shum, E. Lam, K. Wong, K. Tsia. Ultrafast laser-scanning time-stretch imaging at visible wavelengths. Light Sci. Appl., 6, e16196(2017).
[17] M. Hassan, J. Baskin, B. Liao, A. H. Zewail. High-temporal-resolution electron microscopy for imaging ultrafast electron dynamics. Nat. Photon., 11, 425(2017).
[18] K. Sugioka, Y. Cheng. Ultrafast lasers—reliable tools for advanced materials processing. Light Sci. Appl., 3, e149(2014).
[19] G. B. Rieker, F. R. Giorgetta, W. C. Swann, J. Kofler, A. M. Zolot, L. C. Sinclair, E. Baumann, C. Cromer, G. Petron, C. Sweeney, P. P. Tans, I. Coddington, N. R. Newbury. Frequency-comb-based remote sensing of greenhouse gases over kilometer air paths. Optica, 1, 290(2014).
[20] A. Rohrbacher, O. E. Olarte, V. Villamaina, P. L. Alvarez, B. Resan. Multiphoton imaging with blue-diode-pumped SESAM-modelocked Ti:sapphire oscillator generating 5 nJ 82 fs pulses. Opt. Express, 25, 10677(2017).
[21] U. Keller. Recent developments in compact ultrafast lasers. Nature, 424, 831(2003).
[22] O. Okhotnikov, A. Grudinin, M. Pessa. Ultra-fast fiber laser systems based on SESAM technology: new horizons and applications. New J. Phys., 6, 177(2004).
[23] Y. Mao, X. Tong, Z. Wang, L. Zhan, P. Hu, L. Chen. Wavelength-tunable 10 GHz actively harmonic mode-locked fiber laser based on semiconductor optical amplifier. Appl. Phys. B, 121, 517(2015).
[24] A. J. Mercante, S. Shi, P. Yao, L. Xie, R. M. Weikle, D. W. Prather. Thin film lithium niobate electro-optic modulator with terahertz operating bandwidth. Opt. Express, 26, 14810(2018).
[25] J. Qin, R. Dai, Y. Li, Y. Meng, Y. Xu, S. Zhu, F. Wang. 20 GHz actively mode-locked thulium fiber laser. Opt. Express, 26, 25769(2018).
[26] W. H. Glenn, M. J. Brienza, A. J. DeMaria. Mode locking of an organic dye laser. Appl. Phys. Lett., 12, 54(1968).
[27] C. V. Shank, E. P. Ippen. Subpicosecond kilowatt pulses from a mode-locked cw dye laser. Appl. Phys. Lett., 24, 373(1974).
[28] C. Ausschnitt, R. Jain, J. Heritage. Cavity length detuning characteristics of the synchronously mode-locked CW dye laser. IEEE J. Quantum Elect., 15, 912(1979).
[29] S. Vasilyev, M. Mirov, V. Gapontsev. Kerr-lens mode-locked femtosecond polycrystalline Cr2+: ZnS and Cr2+: ZnSe lasers. Opt. Express, 22, 5118(2014).
[30] Z.-Y. Gao, J.-F. Zhu, K. Wang, J.-L. Wang, Z.-H. Wang, Z.-Y. Wei. Diode-pumped Kerr-lens mode-locked femtosecond Yb:YAG ceramic laser. Chin. Phys. B, 25, 024205(2016).
[31] S. Ghanbari, R. Akbari, A. Major. Femtosecond Kerr-lens mode-locked alexandrite laser. Opt. Express, 24, 14836(2016).
[32] U. Keller, K. J. Weingarten, F. X. Kartner, D. Kopf, B. Braun, I. D. Jung, R. Fluck, C. Honninger, N. Matuschek, J. Aus der Au. Semiconductor saturable absorber mirrors (SESAM’s) for femtosecond to nanosecond pulse generation in solid-state lasers. IEEE J. Quantum Electron., 2, 435(1996).
[33] F. Wang, A. Rozhin, V. Scardaci, Z. Sun, F. Hennrich, I. H. White, W. I. Milne, A. C. Ferrari. Wideband-tuneable, nanotube mode-locked, fibre laser. Nat. Nanotechnol., 3, 738(2008).
[34] G. Sobon. “Mode-locking of fiber lasers using novel two-dimensional nanomaterials: graphene and topological insulators [Invited]. Photon. Res., 3, A56(2015).
[35] T. Hasan, Z. Sun, F. Wang, F. Bonaccorso, P. H. Tan, A. G. Rozhin, A. C. Ferrari. Nanotube–polymer composites for ultrafast photonics. Adv. Mater., 21, 3874(2009).
[36] A. Martinez, Z. Sun. Nanotube and graphene saturable absorbers for fibre lasers. Nature Photon., 7, 842(2013).
[37] G. Wang, A. A. Baker-Murray, W. J. Blau. Saturable absorption in 2D nanomaterials and related photonic devices. Laser Photon. Rev., 13, 1800282(2019).
[38] B. Guo, Q. Xiao, S. Wang, H. Zhang. 2D layered materials: synthesis, nonlinear optical properties, and device applications. Laser Photon. Rev., 13, 1800327(2019).
[39] U. Keller, W. H. Knox, H. Roskos. Coupled-cavity resonant passive mode-locked (RPM) Ti:sapphire laser. Opt. Lett., 15, 1377(1990).
[40] I. D. Jung, F. X. Kärtner, N. Matuschek, D. H. Sutter, F. Morier-Genoud, G. Zhang, U. Keller, V. Scheuer, M. Tilsch, T. Tschudi. Self-starting 6.5-fs pulses from a Ti:sapphire laser. Opt. Lett., 22, 1009(1997).
[41] F. X. Kurtner, J. A. der Au, U. Keller. Mode-locking with slow and fast saturable absorbers – what’s the difference?. IEEE J. Sel. Top. Quantum Electron, 4, 159(1998).
[42] R. Paschotta, U. Keller. Passive mode-locking with slow saturable absorbers. Appl. Phys. B, 73, 653(2001).
[43] G. J. Spühler, K.J. Weingarten, R. Grange, L. Krainer, M. Halml, V. Liverini, M. Golling, S. Schon, U. Keller. Semiconductor saturable absorber mirror structures with low saturation fluence. Appl. Phys. B, 81, 27(2005).
[44] C. Hönninger, R. Paschotta, F. Morier-Genoud, M. Moser, U. Keller. Q-switching stability limits of continuous-wave passive mode locking. J. Opt. Soc. Am. B, 16, 46(1999).
[45] S. A. Campbell. The Science and Engineering of Microelectronic Fabrication(2001).
[46] E. U. Rafailov, A. A. Lagatsky, S. A. Zolotovskaya, W. Sibbett. Compact and efficient mode-locked lasers based on QD-SESAMs. Proc. SPIE, 6998, 69980B(2008).
[47] S. Iijima. Synthesis of carbon nanotubes. Nature, 354, 56(1991).
[48] H.-S. P. Wong, D. Akinwande. Carbon Nanotube and Graphene Device Physics(2011).
[49] J. W. G. Wilder, L. C. Venema, A. G. Rinzler, R. E. Smalley, C. Dekker. Electronic structure of atomically resolved carbon nanotubes. Nature, 391, 59(1998).
[50] S. Reich, M. Dworzak, A. Hoffmann, C. Thomsen, M. S. Strano. Excited-state carrier lifetime in single-walled carbon nanotubes. Phys. Rev. B, 71, 033402(2005).
[51] Y.-Z. Ma, J. Stenger, J. Zimmermann, S. M. Bachilo, R. E. Smalley, R. B. Weisman, G. R. Fleming. Ultrafast carrier dynamics in single-walled carbon nanotubes probed by femtosecond spectroscopy. J. Chem. Phys., 120, 3368(2004).
[52] S. Set, H. Yaguchi, M. Jablonski, Y. Tanaka, Y. Sakakibara, A. Rozhin, M. Tokumoto, H. Kataura, Y. Achiba. A noise suppressing saturable absorber at 1550 nm based on carbon nanotube technology, 723.
[53] S. Kivistö, T. Hakulinen, A. Kaskela, B. Aitchison, D. P. Brown, A. G. Nasibulin, E. I. Kauppinen, A. Härkönen, O. Okhotnikov. Carbon nanotube films for ultrafast broadband technology. Opt. Express, 17, 2358(2009).
[54] M. A. Solodyankin, E. D. Obraztsova, A. S. Lobach, A. I. Chernov, A. V. Tausenev, V. Konov, E. M. Dianov. Mode-locked 1.93 µm thulium fiber laser with a carbon nanotube absorber. Opt. Lett., 33, 1336(2008).
[55] L. Huang, Y. Zhang, X. Liu. Dynamics of carbon nanotube-based mode-locking fiber lasers. Nanophotonics, 9, 2731(2020).
[56] C. Wei, Y. Lyu, H. Shi, Z. Kang, H. Zhang, G. Qin, Y. Liu. Mid-infrared Q-switched and mode-locked fiber lasers at 2.87 µm based on carbon nanotube. IEEE J Quantum Electron., 25, 1100206(2019).
[57] R. R. Nair, P. Blake, A. N. Grigorenko, K. S. Novoselov, T. J. Booth, T. Stauber, N. M. R. Peres, A. K. Geim. Fine structure constant defines visual transparency of graphene. Science, 320, 1308(2008).
[58] Z. Sun, T. Hasan, F. Torrisi, D. Popa, G. Privitera, F. Wang, F. Bonaccorso, D. M. Basko, A. C. Ferrari. Graphene mode-locked ultrafast laser. ACS Nano, 4, 803(2010).
[59] G. Sobon, J. Sotor, I. Pasternak, A. Krajewska, W. Strupinski, K. M. Abramski. Multilayer graphene-based saturable absorbers with scalable modulation depth for mode-locked Er- and Tm-doped fiber lasers. Opt. Mater. Express, 5, 2884(2015).
[60] K. Seibert, G. C. Cho, W. Kütt, H. Kurz, D. H. Reitze, J. I. Dadap, H. Ahn, M. C. Downer, A. M. Malvezzi. Femtosecond carrier dynamics in graphite. Phys. Rev. B, 42, 2842(1990).
[61] M. Breusing, C. Ropers, T. Elsaesser. Ultrafast carrier dynamics in graphite. Phys. Rev. Lett., 102, 086809(2009).
[62] Q. Bao, H. Zhang, Y. Wang, Z. Ni, Y. Yan, Z. X. Shen, K. P. Loh, D. Y. Tang. Atomic-layer graphene as a saturable absorber for ultrafast pulsed lasers. Adv. Funct. Mater, 19, 3077(2009).
[63] K. S. Novoselov, A. K. Geim, S. V. Morozov, D. Jiang, Y. Zhang, S. V. Dubonos, I. V. Grigorieva, A. A. Firsov. Electric field effect in atomically thin carbon films. Science, 306, 666(2004).
[64] I. H. Baek, H. W. Lee, S. Bae, B. H. Hong, Y. H. Ahn, D.-I. Yeom, F. Rotermund. Mode-locking of sub-70-fs Ti:sapphire laser by graphene saturable absorber. Appl. Phys. Express, 5, 032701(2013).
[65] H. Zhang, D. Y. Tang, L. M. Zhao, Q. L. Bao, K. P. Loh. Large energy mode locking of an erbium-doped fiber laser with atomic layer graphene. Opt. Express, 17, 17630(2009).
[66] D. I. M. Zen, N. Saidin, S. S. A. Damanhuri, S. W. Harun, H. Ahmad, M. A. Ismail, K. Dimyati, A. Halder, M. C. Paul, S. Das, M. Pal, S. K. Bhadra. Mode-locked thulium bismuth codoped fiber laser using graphene saturable absorber in ring cavity: reply. Appl. Opt., 52, 1226(2013).
[67] G. Zhu, X. Zhu, K. Balakrishnan, R. A. Norwood, N. Peyghambarian. Fe2+: ZnSe and graphene Q-switched singly Ho3+-doped ZBLAN fiber lasers at 3 µm. Opt. Mater. Express, 3, 1365(2013).
[68] A. Malouf, O. Henderson-Sapir, S. Set, S. Yamashita, D. J. Ottaway. Two-photon absorption and saturable absorption of mid-IR in graphene. Appl. Phys. Lett., 114, 091111(2019).
[69] M. Xu, T. Liang, M. Shi, H. Chen. Graphene-like two-dimensional materials. Chem. Rev., 113, 3766(2013).
[70] R. I. Woodward, E. J. R. Kelleher, R. C. T. Howe, G. Hu, F. Torrisi, T. Hasan, S. V. Popov, J. R. Taylor. Tunable Q-switched fiber laser based on saturable edge-state absorption in few-layer molybdenum disulfide (MoS2). Opt. Express, 22, 31113(2014).
[71] K. F. Mak, C. Lee, J. Hone, J. Shan, T. F. Heinz. Atomically thin MoS2: a new direct-gap semiconductor. Phys. Rev. Lett., 105, 136805(2010).
[72] A. Kuc, N. Zibouche, T. Heine. Influence of quantum confinement on the electronic structure of the transition metal sulfide TS2. Phys. Rev. B, 83, 245213(2011).
[73] W. S. Yun, S. W. Han, S. C. Hong, I. G. Kim, J. D. Lee. Thickness and strain effects on electronic structures of transition metal dichalcogenides: 2H-MX2 semiconductors (M = Mo, W; X = S, Se, Te). Phys. Rev. B, 85, 033305(2012).
[74] G. J. Spühler, K. J. Weingarten, R. Grange, L. Krainer, M. Haiml, V. Liverini, M. Golling, S. Schön, U. Keller. Semiconductor saturable absorber mirror structures with low saturation fluence. Appl. Phys. B, 81, 27(2005).
[75] D. J. H. C. Maas, A.-R. Bellancourt, M. Hoffmann, B. Rudin, Y. Barbarin, M. Golling, T. Südmeyer, U. Keller. Growth parameter optimization for fast quantum dot SESAMs. Opt. Express, 16, 18646(2008).
[76] B. W. Tilma, M. Mangold, C. A. Zaugg, S. M. Link, D. Waldburger, A. Klenner, A. S. Mayer, E. Gini, M. Golling, U. Keller. Recent advances in ultrafast semiconductor disk lasers. Light: Sci. Appl., 4, e310(2015).
[77] F. Saltarelli, A. Diebold, I. J. Graumann, C. R. Phillips, U. Keller. Self-phase modulation cancellation in a high-power ultrafast thin-disk laser oscillator. Optica, 5, 1603(2018).
[78] M. Mangold, V. J. Wittwer, C. A. Zaugg, S. M. Link, M. Golling, B. W. Tilma, U. Keller. Femtosecond pulses from a modelocked integrated external-cavity surface emitting laser (MIXSEL). Opt. Express, 21, 24904(2013).
[79] X. Wang, Y. J. Zhu, C. Jiang, Y. X. Guo, X. T. Ge, H. M. Chen, J. Q. Ning, C. C. Zheng, Y. Peng, X. H. Li, Z. Y. Zhang. InAs/GaAs quantum dot semiconductor saturable absorber for controllable dual-wavelength passively Q-switched fiber laser. Opt. Express, 27, 20649(2019).
[80] T. Finke, J. Nürnberg, V. Sichkovskyi, M. Golling, U. Keller, J. P. Reithmaier. Temperature resistant fast InxGa1−xAs/GaAs quantum dot saturable absorber for the epitaxial integration into semiconductor surface emitting lasers. Opt. Express, 28, 20954(2020).
[81] S.-H. Kwona, D. H. Song, I.-S. Kim, D.-K. Ko. Operating characteristics of a SESAM-assisted mode-locked laser oscillator with the location of the SESAM position. Opt. Laser. Technol., 133, 106560(2021).
[82] A. E. H. Oehler, T. Südmeyer, K. J. Weingarten, U. Keller. 100 GHz passively mode-locked Er:Yb:glass laser at 1.5 µm with 1.6-ps pulses. Opt. Express, 16, 21930(2008).
[83] U. Keller. Ultrafast solid-state laser oscillators: a success story for the last 20 years with no end in sight. Appl. Phys. B, 100, 15(2010).
[84] C. J. Saraceno, F. Emaury, C. Schriber, M. Hoffmann, M. Golling, T. Südmeyer, U. Keller. Ultrafast thin-disk laser with 80 µJ pulse energy and 242 W of average power. Opt. Lett., 39, 9(2014).
[85] F. Saltarelli, I. J. Graumann, L. Lang, D. Bauer, C. R. Phillips, U. Keller. Power scaling of ultrafast oscillators: 350-W average-power sub-picosecond thin-disk laser. Opt. Express, 27, 31465(2019).
[86] K. A. Williams, M. G. Thompson, I. H. White. Long-wavelength monolithic mode-locked diode lasers. New J. Phys., 6, 179(2004).
[87] E. A. Avrutin, J. H. Marsh, E. L. Portnoi. Monolithic and multi-gigahertz mode-locked semiconductor lasers: constructions, experiments, models and applications. IEEE Proc. Optoelectron., 147, 251(2000).
[88] U. Keller, A. C. Tropper. Passively modelocked surface-emitting semiconductor lasers. Phys. Rep., 429, 67(2006).
[89] C. G. E. Alfieri, D. Waldburger, M. Golling, U. Keller. High-power sub-300-femtosecond quantum dot semiconductor disk lasers. IEEE Photon. Technol. Lett, 30, 525(2018).
[90] A. Laurain, I. Kilen, J. Hader, A. R. Perez, P. Ludewig, W. Stolz, S. Addamane, G. Balakrishnan, S. W. Koch, J. V. Moloney. Modeling and experimental realization of modelocked VECSEL producing high power sub-100 fs pulses. Appl. Phys. Lett., 113, 121113(2018).
[91] M. Scheller, T. L. Wang, B. Kunert, W. Stolz, S. W. Koch, J. V. Moloney. Passively mode locked VECSEL emitting 682 fs pulses with 5.1 W of average output power. Electron Lett., 48, 588(2012).
[92] D. Lorenser, D. J. H. C. Maas, H. J. Unold, A-R. Bellancourt, B. Rudi, E. Gini, D. Ebling, U. Keller. 50-GHz passively mode-locked surface-emitting semiconductor laser with 100 mW average output power. IEEE J. Quantum Electron., 42, 838(2006).
[93] K. G. Wilcox, A. C. Tropper, H. E. Beere, D. A. Ritchie, B. Kunert, B. Heinen, W. Stolz. 4.35 kW peak power femtosecond pulse mode-locked VECSEL for supercontinuum generation. Opt. Express, 21, 1599(2013).
[94] D. J. H. C. Maas, A-R. Bellancourt, B. Rudin, M. Golling, H. J. Unold, T. Südmeyer, U. Keller. Vertical integration of ultrafast semiconductor lasers. Appl. Phys. B, 88, 493(2007).
[95] M. Mangold, C. A. Zaugg, S. M. Link, M. Golling, B. W. Tilma, U. Keller. Pulse repetition rate scaling from 5 to 100 GHz with a high-power semiconductor disk laser. Opt. Express, 22, 6099(2014).
[96] B. Rudin, V. J. Wittwer, D. J. H. C. Maas, M. Hoffmann, O. D. Sieber, Y. Barbarin, M. Golling, T. Südmeyer, U. Keller. High-power MIXSEL: an integrated ultrafast semiconductor laser with 6.4 W average power. Opt. Express, 18, 27582(2010).
[97] C. G. E. Alfieri, D. Waldburger, J. Nürnberg, M. Golling, U. Keller. Sub-150-fs pulses from an optically pumped broadband mode locked integrated external-cavity surface emitting laser. Opt. Lett., 44, 25(2019).
[98] F. Labaye, M. Gaponenko, V. J. Wittwer, A. Diebold, C. Paradis, N. Modsching, L. Merceron, F. Emaury, I. J. Graumann, C. R. Phillips, C. J. Saraceno, C. Kränkel, U. Keller, T. Südmeyer. Extreme ultraviolet light source at a megahertz repetition rate based on high-harmonic generation inside a mode-locked thin-disk laser oscillator. Opt. Lett., 42, 5170(2017).
[99] A. A. Lagatsky, F. Fusari, S. Calvez, S. V. Kurilchik, V. E. Kisel, N. V. Kuleshov, M. D. Dawson, C. T. A. Brown, W. Sibbett. Femtosecond pulse operation of a Tm,Ho-codoped crystalline laser near 2 µm. Opt. Lett., 35, 172(2010).
[100] V. Aleksandrov, A. Gluth, V. Petrov, I. Buchvarov, G. Steinmeyer, J. Paajaste, S. Suomalainen, A. Härkönen, M. Guina, X. Mateos, F. Díaz, U. Griebner. Mode-locked Tm,Ho:KLu(WO4)2 laser at 2060 nm using InGaSb-based SESAMs. Opt. Express, 23, 4614(2015).
[101] K. Merghem, R. Teissier, G. Aubin, A. M. Monakhov, A. Ramdane, A. N. Baranov. Passive mode locking of a GaSb-based quantum well diode laser emitting at 2.1 µm. Appl. Phys. Lett., 107, 111109(2015).
[102] P. Holl, M. Rattunde, S. Adler, S. Kaspar, W. Bronner, A. Bachle, R. Aidam, J. Wagner. Recent advances in power scaling of GaSb-based semiconductor disk lasers. IEEE J. Sel. Top. Quantum Electron, 21, 324(2015).
[103] Y. Zhao, Y. Wang, X. Zhang, X. Mateos, Z. Pan, P. Loiko, W. Zhou, X. Xu, J. Xu, D. Shen, S. Suomalainen, A. Härkönen, M. Guina, U. Griebner, V. Petrov. 87 fs mode-locked Tm,Ho:CaYAlO4 laser at ∼2043 nm. Opt. Lett., 43, 915(2018).
[104] P. W. Metz, F. Reichert, F. Moglia, S. Müller, D.-T. Marzahl, C. Kränkel, G. Huber. High-power red, orange, and green Pr3+:LiYF4 lasers. Opt. Lett., 39, 3193(2014).
[105] K. Gürel, V. J. Wittwer, M. Hoffmann, C. J. Saraceno, S. Hakobyan, B. Resan, A. Rohrbacher, K. Weingarten, S. Schilt, T. Südmeyer. Green-diode-pumped femtosecond Ti:sapphire laser with up to 450 mW average power. Opt. Express, 23, 30043(2015).
[106] Y. Zhang, V. Petrov, U. Griebner, X. Zhang, H. Yu, H. Zhang, J. Liu. Diode-pumped SESAM mode-locked Yb:CLNGG laser,”. Opt. Laser Technol., 69, 144(2015).
[107] J. Ma, Z. Pan, J. Wang, H. Yuan, H. Cai, G. Xie, L. Qian, D. Shen, D. Tang. Generation of sub-50fs soliton pulses from a mode-locked Yb, Na:CNGG disordered crystal laser. Opt. Express, 25, 14968(2017).
[108] A. S. Mayer, C. R. Phillips, U. Keller. Watt-level 10-gigahertz solid-state laser enabled by self-defocusing nonlinearities in an aperiodically poled crystal. Nat. Commun., 8, 1673(2017).
[109] R. Akbari, K. A. Fedorova, E. U. Rafailov, A. Major. Diode-pumped ultrafast Yb:KGW laser with 56 fs pulses and multi-100 kW peak power based on SESAM and Kerr-lens mode locking. Appl. Phys. B, 123, 123(2017).
[110] R. Grange, S. Zeller, M. Haiml, O. Ostinelli, E. Gini, S. Schon, U. Keller. Antimonide semiconductor saturable absorber for passive mode locking of a 1.5-µm Er:Yb:glass laser at 10 GHz. IEEE Photon. Technol. Lett, 18, 805(2006).
[111] A. Choudhary, A. A. Lagatsky, Z. Y. Zhang, K. J. Zhou, Q. Wang, R. A. Hogg, K. Pradeesh, E. U. Rafailov, W. Sibbett, C. T. A. Brown, D. P. Shepherd. A diode-pumped 1.5 µm waveguide laser mode-locked at 6.8 GHz by a quantum dot SESAM. Laser Phys. Lett., 10, 105803(2013).
[112] N. K. Stevenson, C. T. A. Brown, J.-M. Hopkins, M. D. Dawson, C. Kränkel, A. A. Lagatsky. Diode-pumped femtosecond Tm3+-doped LuScO3 laser near 2.1 µm. Opt. Lett., 43, 1287(2018).
[113] X. Bu, Y. Shi, J. Xu, H. Li, P. Wang. 408-fs SESAM mode locked Cr:ZnSe laser. Proc. SPIE, 10619, 1061903(2018).
[114] Y. Wang, W. Jing, P. Loiko, Y. Zhao, H. Huang, X. Mateos, S. Suomalainen, A. Härkönen, M. Guina, U. Griebner, V. Petrov. Sub-10 optical-cycle passively mode-locked Tm:(Lu2/3Sc1/3)2O3 ceramic laser at 2 µm. Opt. Express, 26, 10299(2018).
[115] Y. Wang, Y. Zhao, Z. Pan, S. Suomalainen, A. Härkönen, M. Guina, U. Griebner, L. Wang, P. Loiko, X. Mateos, W. Chen, V. Petrov. 73-fs SESAM mode-locked Tm,Ho:CNGG laser at 2061 nm. Proc. SPIE, 11259, 1125929(2020).
[116] E. Sorokin, I. T. Sorokina. Femtosecond operation and random quasi-phase-matched self-doubling of ceramic Cr:ZnSe laser, CTuGG2(2010).
[117] E. Sorokin, N. Tolstik, K. I. Schaffers, I. T. Sorokina. Femtosecond SESAM-mode locked Cr:ZnS laser. Opt. Express, 20, 28947(2012).
[118] I. J. Graumann, A. Diebold, C. G. E. Alfieri, F. Emaury, B. Deppe, M. Golling, D. Bauer, D. Sutter, C. Kränkel, C. J. Saraceno, C. R. Phillips, U. Keller. Peak-power scaling of femtosecond Yb:Lu2O3 thin-disk lasers. Opt. Express, 25, 22519(2017).
[119] F. Saltarelli, A. Diebold, I. J. Graumann, C. R. Phillips, U. Keller. Self-phase modulation cancellation in a high-power ultrafast thin-disk laser oscillator. Opt. Lett., 5, 25(2018).
[120] A. Härkönen, S. Suomalainen, A. Rantamäki, J. Nikkinen, Y. Wang, U. Griebner, G. Steinmeyer, M. Guina. 1.34 µm VECSEL mode-locked with a GaSb-based SESAM. Opt. Lett., 43, 3353(2018).
[121] T. R. Schibli, K. Minoshima, H. Kataura, E. Itoga, N. Minami, S. Kazaoui, K. Miyashita, M. Tokumoto, Y. Sakakibara. Ultrashort pulse-generation by saturable absorber mirrors based on polymer-embedded carbon nanotubes. Opt. Express, 13, 8025(2005).
[122] D. V. Khudyakov, A. S. Lobach, V. A. Nadtochenko. Passive mode locking in a Ti:sapphire laser using a single-walled carbon nanotube saturable absorber at a wavelength of 810 nm. Opt. Lett., 35, 2675(2010).
[123] I. H. Baek, S. Y. Choi, H. W. Lee, W. B. Cho, V. Petrov, A. Agnesi, V. Pasiskevicius, D. Yeom, K. Kim, F. Rotermund. Single-walled carbon nanotube saturable absorber assisted high-power mode-locking of a Ti:sapphire laser. Opt. Express, 19, 7833(2011).
[124] A. Schmidt, S. Rivier, G. Steinmeyer, J. H. Yim, W. B. Cho, S. Lee, F. Rotermund, M. C. Pujol, X. Mateos, M. Aguiló, F. Díaz, V. Petrov, U. Griebner. Passive mode locking of Yb:KLuW using a single-walled carbon nanotube saturable absorber. Opt. Lett., 33, 729(2008).
[125] S. Y. Choi, T. Calmano, F. Rotermund, C. Kränkel. 2-GHz carbon nanotube mode-locked Yb:YAG channel waveguide laser. Opt. Express, 26, 5140(2018).
[126] W. B. Cho, J. H. Yim, S. Y. Choi, S. Lee, U. Griebner, V. Petrov, F. Rotermund. Mode-locked self-starting Cr:forsterite laser using a single-walled carbon nanotube saturable absorber. Opt. Lett., 33, 2449(2008).
[127] Z. Pan, Y. Wang, Y. Zhao, H. Yuan, X. Dai, H. Cai, J. E. Bae, S. Y. Choi, F. Rotermund, X. Mateos, J. M. Serres, P. Loiko, U. Griebner, V. Petrov. Generation of 84-fs pulses from a mode-locked Tm:CNNGG disordered garnet crystal laser. Photon. Res., 6, 800(2018).
[128] N. Tolstik, O. Okhotnikov, E. Sorokin, I. T. Sorokina. Femtosecond Cr:ZnS laser at 2.35 µm mode-locked by carbon nanotubes. Proc. SPIE, 8959, 89591A(2014).
[129] W. B. Cho, A. Schmidt, J. H. Yim, S. Y. Choi, S. Lee, F. Rotermund, U. Griebner, G. Steinmeyer, V. Petrov, X. Mateos, M. C. Pujol, J. J. Carvajal, M. Aguiló, F. Díaz. Passive mode-locking of a Tm-doped bulk laser near 2 µm using a carbon nanotube saturable absorber. Opt. Express, 17, 11007(2009).
[130] J. Ma, G. Xie, P. Lv, W. Gao, P. Yuan, L. Qian, U. Griebner, V. Petrov, H. Yu, H. Zhang, J. Wang. Wavelength-versatile graphene-gold film saturable absorber mirror for ultra-broadband mode-locking of bulk lasers. Sci. Rep., 4, 5016(2014).
[131] A. G. Khrimchuk, P. A. Obraztsov. 11-GHz waveguide Nd:YAG laser CW mode-locked with single-layer graphene. Sci. Rep., 5, 11172(2015).
[132] N. Tolstik, E. Sorokin, I. T. Sorokina. Graphene mode-locked Cr:ZnS laser with 41 fs pulse duration. Opt. Express, 22, 5564(2014).
[133] C. Feng, X. Zhang, J. Wang, Z. Liu, Z. Cong, H. Rao, Q. Wang, J. Fang. Passively mode-locked Nd3+:YVO4 laser using a molybdenum disulfide as saturable absorber. Opt. Mater. Express, 6, 1358(2016).
[134] L. Tao, X. Huang, J. He, Y. Lou, L. Zeng, Y. Li, H. Long, J. Li, L. Zhang, Y. H. Tsang. Vertically standing PtSe2 film: a saturable absorber for a passively mode-locked Nd:LuVO4 laser. Photon. Res., 6, 750(2018).
[135] K. Seger, N. Meiser, S. Y. Choi, B. H. Jung, D.-I. Yeom, F. Rotermund, O. Okhotnikov, F. Laurell, V. Pasiskevicius. Carbon nanotube mode-locked optically-pumped semiconductor disk laser. Opt. Express, 21, 17806(2013).
[136] C. A. Zaugg, Z. Sun, V. J. Wittwer, D. Popa, S. Milana, T. S. Kulmala, R. S. Sundaram, M. Mangold, O. D. Sieber, M. Golling, Y. Lee, J. H. Ahn, A. C. Ferrari, U. Keller. Ultrafast and widely tuneable vertical-external-cavity surface-emitting laser, mode-locked by a graphene-integrated distributed Bragg reflector. Opt. Express, 21, 31548(2013).
[137] S. Husaini, R. G. Bedford. Graphene saturable absorber for high power semiconductor disk laser mode-locking. Appl. Phys. Lett., 104, 161107(2014).
[138] M. S. Gaponenko, V. E. Kisel, N. V. Kuleshov, A. M. Malyarevich, K. V. Yumashev, A. A. Onushchenko. Compact passively Q-switched diode-pumped Tm:KY(WO4)2 laser with 8 ns/30 µJ pulses. Laser Phys. Lett., 7, 286(2010).
[139] M. Zhang, R. C. T. Howe, R. I. Edmund, J. R. Kelleher, F. Torrisi, G. Hu, S. V. Popov, J. R. Taylor, T. Hasan. Solution processed MoS2-PVA composite for sub-bandgap mode-locking of a wideband tunable ultrafast Er:fiber laser. Nano Res., 8, 1522(2015).
[140] M. Yi, Z. Shen. A review on mechanical exfoliation for the scalable production of graphene. J. Mater. Chem. A, 3, 11700(2015).
[141] H. Zhang, S. B. Lu, J. Zheng, J. Du, S. C. Wen, D. Y. Tang, K. P. Loh. Molybdenum disulfide (MoS2) as a broadband saturable absorber for ultra-fast photonics. Opt. Express, 22, 7249(2014).
[142] D. Steinberg, R. M. Gerosa, F. N. Pellicer, J. D. Zapata, S. H. Domingues, E. A. Thoroh de Souza, L. A. M. Saito. Graphene oxide and reduced graphene oxide as saturable absorbers onto D-shaped fibers for sub 200-fs EDFL mode-locking. Opt. Mater. Express, 8, 144(2018).
[143] J. N. Coleman, M. Lotya, A. O’Neill, S. D. Bergin, P. J. King, U. Khan, V. Nicolosi. Two-dimensional nanosheets produced by liquid exfoliation of layered materials. Science, 331, 568(2011).
[144] T. Jiang, K. Yin, C. Wang, J. You, H. Ouyang, R. Miao, C. Zhang, K. Wei, H. Li, H. Chen, R. Zhang, X. Zheng, Z. Xu, X. Cheng, H. Zhang. Ultrafast fiber lasers mode-locked by two-dimensional materials: review and prospect. Photon. Res., 8, 78(2020).
[145] D. Popa, Z. Sun, T. Hasan, W. B. Cho, F. Wang, F. Torrisi, A. C. Ferrari. 74-fs nanotube-mode-locked fiber laser. Appl. Phys. Lett., 101, 153107(2012).
[146] D. Mao, B. Jiang, W. Zhang, J. Zhao. Pulse-state switchable fiber laser mode-locked by carbon nanotubes. IEEE Photon. Technol. Lett., 27, 253(2015).
[147] Y. Meng, Y. Li, Y. Xu, F. Wang. Carbon nanotube mode-locked thulium fiber laser with 200 nm tuning range. Sci. Rep., 7, 45109(2017).
[148] J. W. Nicholson, R. S. Windeler, D. J. DiGiovanni. Optically driven deposition of single-walled carbon-nanotube saturable absorbers on optical fiber end-faces. Opt. Express, 15, 9176(2007).
[149] G. Sobon, A. Duzynska, M. Świniarski, J. Judek, J. Sotor, M. Zdrojek. CNT-based saturable absorbers with scalable modulation depth for thulium-doped fiber lasers operating at 1.9 µm. Sci. Rep., 7, 45491(2017).
[150] W. Liu, M. Liu, X. Chen, T. Shen, M. Lei, J. Guo, H. Deng, W. Zhang, C. Dai, X. Zhang, Z. Wei. Ultrafast photonics of two dimensional AuTe2Se4/3 in fiber lasers. Commun. Phys., 3, 15(2020).
[151] K. Kieu, M. Mansuripur. Femtosecond laser pulse generation with a fiber taper embedded in carbon nanotube/polymer composite. Opt. Lett., 32, 2242(2007).
[152] Y.-W. Song, S. Yamashita, S. Maruyama. Single-walled carbon nanotubes for high-energy optical pulse formation. Appl. Phys. Lett., 92, 021115(2008).
[153] M. Liu, X.-W. Zheng, Y.-L. Qi, H. Liu, A.-P. Luo, Z.-C. Luo, W.-C. Xu, C.-J. Zhao, H. Zhang. Microfiber-based few-layer MoS2 saturable absorber for 2.5 GHz passively harmonic mode-locked fiber laser. Opt. Express, 22, 22841(2014).
[154] M. Zhang, J. Li, H. Chen, J. Zhang, J. Yin, T. He, J. Wang, M. Zhang, B. Zhang, J. Yuan, P. Yan, S. Ruan. Group IIIA/IVA monochalcogenides nanosheets for ultrafast photonics. APL Photon., 4, 090801(2019).
[155] J. D. Yin, F. X. Zhu, J. T. Lai, H. Chen, M. Y. Zhang, J. Q. Zhang, J. T. Wang, T. C. He, B. Zhang, J. P. Yuan, P. G. Yan, S. C. Ruan. Hafnium sulfide nanosheets for ultrafast photonic device. Adv. Opt. Mater., 7, 1801303(2018).
[156] X. Wu, Z. W. Zhou, J. D. Yin, M. Zhang, L. L. Zhou, Q. X. Na, J. T. Wang, Y. Yu, J. B. Yang, R. H. Chi. Ultrafast fiber laser based on HfSe2 saturable absorber. Nanotechonlogy, 31, 24(2020).
[157] X. Xu, M. He, C. Quan, R. Wang, C. Liu, Q. Zhao, Y. Zhou, J. Bai, X. Xu. Saturable absorption properties of ReS2 films and mode-locking application based on double-covered ReS2 micro fiber. J. Lightwave Technol., 36, 5130(2018).
[158] G. Hu, L. Yang, Z. Yang, Y. Wang, X. Jin, J. Dai, Q. Wu, S. Liu, X. Zhu, X. Wang, T. Wu, R. C. T. Howe, T. Albrow-Owen, L. W. T. Ng, Q. Yang, L. G. Occhipinti, R. I. Woodward, E. J. R. Kelleher, Z. Sun, X. Huang, M. Zhang, C. D. Bain, T. Hasan. A general ink formulation of 2D crystals for wafer-scale inkjet printing. Sci. Adv., 6, eaba5029(2020).
[159] Y. Gao, W. Shi, W. Wang, Y. Leng, Y. Zhao. Inkjet printing patterns of highly conductive pristine graphene on flexible substrates. Ind. Eng. Chem. Res., 53, 16777(2014).
[160] Y. I. Jhon, J. Koo, B. Anasori, M. Seo, J. Lee, Y. Gogotsi, Y. M. Jhon. 2D materials: metallic MXene saturable absorber for femtosecond mode-locked lasers. Adv Mater., 29, 1702496(2017).
[161] G. Hu, T. A. Owen, X. Jin, A. Ali, Y. Hu, R. C. T. Howe, K. Shehzad, Z. Yang, X. Zhu, R. I. Woodward, T.-C. Wu, H. Jussila, J.-B. Wu, P. Peng, P.-H. Tan, Z. Sun, E. J. R. Kelleher, M. Zhang, Y. Xu, T. Hasan. Black phosphorus ink formulation for inkjet printing of optoelectronics and photonics. Nat. Commun., 8, 1(2017).
[162] X. Jin, G. Hu, M. Zhang, Y. Hu, T. A. Owen, R. C. T. Howe, T.-C. Wu, Q. Wu, Z. Zheng, T. Hasan. 102 fs pulse generation from a long-term stable, inkjet-printed black phosphorus-mode-locked fiber laser. Opt. Express, 26, 12506(2018).
[163] X. Jiang, W. Li, T. Hai, R. Yue, Z. Chen, C. Lao, Y. Ge, G. Xie, Q. Wen, H. Zhang. Inkjet-printed MXene micro-scale devices for integrated broadband ultrafast photonics. NPJ 2D Mater. Appl., 3, 34(2019).
[164] J. Lee, H. Chung, J. Koo, G. Woo, J. H. Lee. A 3-D printed saturable absorber for femtosecond mode-locking of a fiber laser. Opt. Mater., 89, 382(2019).
[165] G. Woo, J. Lee, J. H. Lee. A 1.9 µm femtosecond fiber laser using a 3D printed, all-fiberized graphene/polylactic-acid saturable absorber. Laser Phys. Lett, 16, 085101(2019).
[166] K. Kieu, F. W. Wise. All-fiber normal-dispersion femtosecond laser. Opt. Express, 16, 11453(2008).
[167] L. Hou, H. Guo, Y. Wang, J. Sun, Q. Lin, Y. Bai, J. Bai. Sub-200 femtosecond dispersion-managed soliton ytterbium-doped fiber laser based on carbon nanotubes saturable absorber. Opt. Express, 26, 9063(2018).
[168] G. Sobon, A. Duzynska, M. Świniarski, J. Judek, J. Sotor, M. Zdrojek. CNT-based saturable absorbers with scalable modulation depth for thulium-doped fiber lasers operating at 1.9 µm. Sci. Rep., 7, 45491(2017).
[169] Z. Sun, T. Hasan, F. Wang, A. G. Rozhin, I. H. White, A. C. Ferrari. Ultrafast stretched-pulse fiber laser mode-locked by carbon nanotubes. Nano Res., 3, 404(2010).
[170] A. Martinez, S. Yamashita. Multi-gigahertz repetition rate passively mode locked fiber lasers using carbon nanotubes. Opt. Express, 19, 6155(2011).
[171] J. Wang, X. Liang, G. Hu, Z. Zheng, S. Lin, D. Ouyang, X. Wu, P. Yan, S. Ruan, Z. Sun, T. Hasan. 152 fs nanotube-mode-locked thulium-doped all-fiber laser. Sci. Rep., 6, 28885(2016).
[172] Z. Sun, T. Hasan, F. Torrisi, D. Popa, G. Privitera, F. Wang, F. Bonaorso, D. M. Basko, A. C. Ferrari. Graphene mode-locked ultrafast laser. ACS Nano, 4, 803(2010).
[173] D. G. Purdie, D. Popa, V. J. Wittwer, Z. Jiang, G. Bonacchini, F. Torrisi, S. Milana, E. Lidorikis, A. C. Ferrari. Few-cycle pulses from a graphene mode-locked all-fiber laser. Appl. Phys. Lett., 106, 253101(2015).
[174] D. Popa, Z. Sun, F. Torrisi, T. Hasan, F. Wang, A. C. Ferrari. Sub 200 fs pulse generation from a graphene mode-locked fiber laser. Appl. Phys. Lett., 97, 203106(2010).
[175] A. Martinez, S. Yamashita. 10 GHz fundamental mode fiber laser using a graphene saturable absorber. Appl. Phys. Lett., 101, 041118(2012).
[176] Y. Zhang, J. Zhu, P. Li, X. Wang, H. Yu, K. Xiao, C. Li, G. Zhang. All-fiber Yb-doped fiber laser passively mode-locking by monolayer MoS2 saturable absorber. Opt. Commun., 413, 236(2018).
[177] H. Liu, A.-P. Luo, F.-Z. Wang, R. Tang, M. Liu, Z.-C. Luo, W.-C. Xu, C.-J. Zhao, H. Zhang. Femtosecond pulse erbium-doped fiber laser by a few-layer MoS2 saturable absorber,”. Opt. Lett., 39, 4591(2014).
[178] K. Wu, X. Zhang, J. Wang, X. Li, J. Chen. WS2 as a saturable absorber for ultrafast photonic applications of mode-locked and Q-switched lasers. Opt. Express, 23, 11453(2015).
[179] L. Li, Y. Su, Y. Wang, X. Wang, Y. Wang, X. Li, D. Mao, J. Si. Femtosecond passively Er-doped mode-locked fiber laser with WS2 solution saturable absorber. IEEE J. Quantum. Electron., 23, 44(2017).
[180] R. Khazaeinezhad, S. H. Kassani, H. Jeong, D.-I Yeom, K. Oh. Femtosecond soliton pulse generation using evanescent field interaction through tungsten disulfide (WS2) film. IEEE J. Lightwave Technol., 33, 3550(2015).
[181] R. Khazaeinezhad, S. H. Kassani, H. Jeong, K. J. Park, B. Yoon Kim, D.-I. Yeom, K. Oh. Ultrafast pulsed all-fiber laser based on tapered fiber enclosed by few-layer WS2 nanosheets. IEEE Photon. Technol. Lett, 27, 1581(2015).
[182] D. Mao, X. She, B. Du, D. Yang, W. Zhang, K. Song, X. Cui, B. Jiang, T. Peng, J. Zhao. Erbium-doped fiber laser passively mode locked with few-layer WSe2/MoSe2 nanosheets. Sci. Rep., 6, 23583(2016).
[183] H. Ahmad, S. N. Aidit, N. A. Hassan, M. F. Ismail, Z. C. Tiu. Generation of mode-locked erbium-doped fiber laser using MoSe2 as saturable absorber. Opt. Lasers Eng., 55, 076115(2016).
[184] D. Mao, X. Cui, X. Gan, M. Li, W. Zhang, H. Lu, J. Zhao. Passively Q-switched and mode-locked fiber laser based on an ReS2 saturable absorber. IEEE J. Quantum Electron., 24, 1100406(2018).
[185] K. Niu, R. Sun, Q. Chen, B. Man, H. Zhang. Passively mode-locked Er-doped fiber laser based on SnS2 nanosheets as a saturable absorber. Photon. Res., 6, 72(2018).
[186] D. Mao, B. Jiang, X. Gan, C. Ma, Y. Chen, C. Zhao, H. Zhang, J. Zheng, J. Zhao. Soliton fiber laser mode locked with two types of film-based Bi2Te3 saturable absorbers. Photon. Res., 3, A43(2015).
[187] H. Liu, X.-W. Zheng, M. Liu, N. Zhao, A.-P. Luo, Z.-C. Luo, W.-C. Xu, H. Zhang, C.-J. Zhao, S.-C. Wen. Femtosecond pulse generation from a topological insulator mode-locked fiber laser. Opt. Express, 22, 6868(2014).
[188] L. Jin, X. Ma, H. Zhang, H. Zhang, H. Chen, Y. Xu. 3 GHz passively harmonic mode-locked Er-doped fiber laser by evanescent field-based nano-sheets topological insulator. Opt. Express, 26, 31244(2018).
[189] B. Guo, S.-H. Wang, Z.-X. Wu, Z.-X. Wang, D.-H. Wang, H. Huang, F. Zhang, Y.-Q. Ge, H. Zhang. Sub-200 fs soliton mode-locked fiber laser based on bismuthene saturable absorber. Opt. Express, 26, 22750(2018).
[190] J. Sotor, G. Sobon, W. Macherzynski, P. Paletko, K. M. Abramski. Black phosphorus saturable absorber for ultrashort pulse generation. Appl. Phys. Lett., 107, 051108(2015).
[191] Y. Ge, Z. Zhu, Y. Xu, Y. Chen, S. Chen, Z. Liang, Y. Song, Y. Zou, H. Zeng, S. Xu, H. Zhang, D. Fan. Broadband nonlinear photoresponse of 2D TiS2 for ultrashort pulse generation and all-optical thresholding devices. Adv. Opt. Mater., 6, 1701166(2017).
[192] P. Yan, R. Lin, S. Ruan, A. Liu, H. Chen, Y. Zheng, S. Chen, C. Guo, J. Hu. A practical topological insulator saturable absorber for mode-locked fiber laser. Sci. Rep., 5, 8690(2015).
[193] P. Yan, R. Lin, S. Ruan, A. Liu, H. Chen. A 2.95 GHz, femtosecond passive harmonic mode-locked fiber laser based on evanescent field interaction with topological insulator film. Opt. Express, 23, 154(2015).
[194] J. Boguslawski, G. Sobon, R. Zybala, J. Sotor. Dissipative soliton generation in Er-doped fiber laser mode-locked by Sb2Te3 topological insulator. Opt. Lett., 40, 2786(2015).
[195] J. Wang, J. Yin, T. He, P. Yan. Sb2Te3 mode-locked ultrafast fiber laser at 1.93 µm. Chin. Phys. B, 27, 084214(2018).
[196] J. Wang, Z. Jiang, H. Chen, J. Li, J. Yin, J. Wang, T. He, P. Yan, S. Ruan. High energy soliton pulse generation by a magnetron-sputtering-deposition-grown MoTe2 saturable absorber. Photon. Res., 6, 535(2018).
[197] J. Wang, Z. Jiang, H. Chen, J. Li, J. Yin, J. Wang, T. He, P. Yan, S. Ruan. Magnetron-sputtering deposited WTe2 for an ultrafast thulium-doped fiber laser. Opt. Lett., 42, 5010(2017).
[198] P. Yan, H. Chen, A. Liu, K. Li, S. Ruan, J. Ding, X. Qiu, T. Guo. Self-starting mode-locking by fiber-integrated WS2 saturable absorber mirror. IEEE J. Quantum Electron., 23, 33(2017).
[199] H. Chen, J. Yin, J. Yang, X. Zhang, M. Liu, Z. Jiang, J. Wang, Z. Sun, T. Guo, W. Liu, P. Yan. Transition-metal dichalcogenides heterostructure saturable absorbers for ultrafast photonics. Opt. Lett., 42, 4279(2017).
[200] W. Liu, L. Pang, H. Han, W. Tian, H. Chen, M. Lei, P. Yan, Z. Wei. 70-fs mode-locked erbium-doped fiber laser with topological insulator. Sci. Rep., 6, 19997(2016).
[201] M. Kowalczyk, J. Bogusławski, R. Zybała, K. Mars, A. Mikuła, G. Soboń, J. Sotor. Sb2Te3-deposited D-shaped fiber as a saturable absorber for mode-locked Yb-doped fiber lasers. Opt. Mater. Express, 6, 2273(2016).
[202] P. Yan, Z. Jiang, H. Chen, J. Yin, J. Lai, J. Wang, T. He, J. Yang. α-In2Se3 wideband optical modulator for pulsed fiber lasers. Opt. Lett., 43, 4417(2018).
[203] P. Yan, A. Liu, Y. Chen, H. Chen, S. Ruan, C. Guo, S. Chen, I. L. Li, H. Yang, J. Hu, G. Cao. Microfiber-based WS2-film saturable absorber for ultra-fast photonics. Opt. Mater. Express, 5, 479(2015).
[204] W. Liu, Y.-N. Zhu, M. Liu, B. Wen, S. Fang, H. Teng, M. Lei, L.-M. Liu, Z. Wei. Optical properties and applications for MoS2-Sb2Te3-MoS2 heterostructure materials. Photon. Res., 6, 227(2018).
[205] Y. Meng, C. Zhu, Y. Li, X. Yuan, F. Xiu, Y. Shi, Y. Xu, F. Wang. Three-dimensional Dirac semimetal thin-film absorber for broadband pulse generation in the near-infrared. Opt. Lett., 43, 1503(2018).
[206] J. Sotor, I. Pasternak, A. Krajewska, W. Strupinski, G. Sobon. Sub-90 fs a stretched-pulse mode-locked fiber laser based on a graphene saturable absorber. Opt. Express, 23, 27503(2015).
[207] G. Sobon, J. Sotor, I. Pasternak, A. Krajewska, W. Strupinski, K. M. Abramski. Thulium-doped all-fiber laser mode-locked by CVD-graphene/PMMA saturable absorber. Opt. Express, 21, 12797(2013).
[208] X. Wu, S. Yu, H. Yang, W. Li, X. Liu, L. Tong. Effective transfer of micron-size graphene to microfibers for photonic applications. Carbon, 96, 1114(2016).
[209] H. Xia, H. Li, C. Lan, C. Li, X. Zhang, S. Zhang, Y. Liu. Ultrafast erbium-doped fiber laser mode-locked by a CVD-grown molybdenum disulfide (MoS2) saturable absorber. Opt. Express, 22, 17341(2014).
[210] P. Yan, H. Chen, J. Yin, Z. Xu, J. Li, Z. Jiang, W. Zhang, J. Wang, I. L. Li, Z. Sun, S. Ruan. Large-area tungsten disulfide for ultrafast photonics. Nanoscale, 9, 1871(2017).
[211] R. Khazaeizhad, S. H. Kassani, H. Jeong, D. Yeom, K. Oh. Passively mode-locked fiber laser based on CVD WS2, JW2A.74(2015).
[212] W. Liu, M. Liu, Y. O. Yang, H. Hou, M. Lei, Z. Wei. CVD-grown MoSe2 with high modulation depth for ultrafast mode-locked erbium-doped fiber laser. Nanotechnology, 29, 394002(2018).
[213] W. Liu, M. Liu, J. Yin, H. Chen, W. Lu, S. Fang, H. Teng, M. Lei, P. Yan, Z. Wei. Tungsten diselenide for all-fiber lasers with the chemical vapor deposition method. Nanoscale, 10, 7971(2018).
[214] J. Wang, W. Lu, J. Li, H. Chen, Z. Jiang, J. Wang, W. Zhang, M. Zhang, I. L. Li, Z. Xu, W. Liu, P. Yan. Ultrafast thulium-doped fiber laser mode locked by monolayer WSe2. IEEE J. Quantum Electron., 24, 1100706(2018).
[215] J. Yin, J. Li, H. Chen, J. Wang, P. Yan, M. Liu, W. Liu, W. Lu, Z. Xu, W. Zhang, J. Wang, Z. Sun, S. Ruan. Large-area highly crystalline WSe2 atomic layers for ultrafast pulsed lasers. Opt. Express, 25, 30020(2017).
[216] J. Wang, H. Chen, Z. Jiang, J. Yin, J. Wang, M. Zhang, T. He, J. Li, P. Yan, S. Ruan. Mode-locked thulium-doped fiber laser with chemical vapor deposited molybdenum ditelluride. Opt. Lett., 43, 1998(2018).
[217] K. Zhang, M. Feng, Y. Ren, F. Liu, X. Chen, J. Yang, X.-Q. Yan, F. Song, J. Tian. Q-switched and mode-locked Er-doped fiber laser using PtSe2 as a saturable absorber. Photon. Res., 6, 893(2018).
[218] Q. Guo, J. Pan, Y. Liu, H. Si, Z. Lu, X. Han, J. Gao, Z. Zuo, H. Zhang, S. Jiang. Output energy enhancement in a mode-locked Er-doped fiber laser using CVD-Bi2Se3 as a saturable absorber. Opt. Express, 27, 24670(2019).
[219] G. Zhu, X. Zhu, F. Wang, S. Xu, Y. Li, X. Guo, K. Balakrishnan, R. A. Norwood, N. Peyghambarian. Graphene mode-locked fiber laser at 2.8 µm. IEEE Photon. Technol. Lett, 28, 7(2016).
[220] K. Yin, T. Jiang, H. Yu, X. Zheng, X. Cheng, J. Hou. Mid-infrared ultra-short mode-locked fiber laser utilizing topological insulator Bi2Te3 nano-sheets as the saturable absorber(2015).
[221] Z. Qin, G. Xie, C. Zhao, S. Wen, P. Yuan, L. Qian. Mid-infrared mode-locked pulse generation with multilayer black phosphorus as saturable absorber. Opt. Lett., 41, 56(2015).
[222] T. Hu, D. D. Hudson, S. D. Jackson. Stable, self-starting, passively mode-locked fiber ring laser of the 3 µm class. Opt. Lett., 39, 2133(2014).
[223] Z. Qin, G. Xie, J. Ma, P. Yuan, L. Qian. 2.8 µm all-fiber Q-switched and mode-locked lasers with black phosphorus. Photon. Res., 6, 1074(2018).
[224] Z. Qin, T. Hai, G. Xie, J. Ma, P. Yuan, L. Qian, L. Li, L. Zhao, D. Shen. Black phosphorus Q-switched and mode-locked mid-infrared Er:ZBLAN fiber laser at 3.5 µm wavelength. Opt. Express, 26, 8224(2018).
[225] Z. Qin, G. Xie, H. Gu, T. Hai, P. Yuan, J. Ma, L. Qian. Mode-locked 2.8-µm fluoride fiber laser: from soliton to breathing pulse. Adv. Photon., 1, 065001(2019).
[226] W. Du, H. Li, C. Lan, C. Li, J. Li, Z. Wang, Y. Liu. Graphene/WS2 heterostructure saturable absorbers for ultrashort pulse generation in L-band passively mode-locked fiber lasers. Opt. Express, 28, 11514(2020).
[227] X. Hong, J. Kim, S.-F. Shi, Y. Zhang, C. Jin, Y. Sun, S. Tongay, J. Wu, Y. Zhang, F. Wang. Ultrafast charge transfer in atomically thin MoS2/WS2 heterostructures. Nat. Nanotech., 9, 682(2014).
[228] Y. Yu, S. Hu, L. Su, L. Huang, Y. Liu, Z. Jin, A. A. Purezky, D. B. Geohegan, K. W. Kim, Y. Zhang, L. Cao. Equally efficient interlayer exciton relaxation and improved absorption in epitaxial and nonepitaxial MoS2/WS2 heterostructures. Nano Lett., 15, 486(2014).
[229] K. S. Novoselov, A. Mishchenko, A. Carvalho, A. H. Castro Neto. 2D materials and van der Waals heterostructures. Science, 353, aac9439(2016).
[230] J. Wang, Z. Li, H. Chen, G. Deng, X. Niu. Recent advances in 2D lateral heterostructures. Nano-Micro Lett., 11, 48(2019).
[231] W. J. Liu, M. L. Liu, B. Liu, R. G. Quhe, M. Lei, S. B. Fang, H. Teng, Z. Y. Wei. Nonlinear optical properties of MoS2-WS2 heterostructure in fiber lasers. Opt. Express, 27, 6689(2019).
[232] Z. Wang, H. Mu, J. Yuan, C. Zhao, Q. Bao, H. Zhang. Graphene–Bi2Te3 heterostructure as saturable absorber for short pulse generation. ACS Photon., 9, 832(2015).
[233] Y. Wang, H. Mu, X. Li, J. Yuan, J. Chen, S. Xiao, Q. Bao, Y. Gao, J. He. Observation of large nonlinear responses in a graphene-Bi2Te3 heterostructure at a telecommunication wavelength. Appl. Phys. Lett., 108, 221901(2016).
[234] J. Wang, A. Coillet, O. Demichel, Z. Wang, D. Rego, A. Bouhelier, P. Grelu, B. Cluzel. Saturable plasmonic metasurfaces for laser mode locking. Light Sci. Appl., 9, 50(2020).
[235] E. J. Lee, S. Y. Choi, H. Jeong, N. H. Park, W. Yim, M. H. Kim, J-K Park, S. Son, S. Bae, S. J. Kim, K. Lee, Y. H. Ahn, K. J. Ahn, B. H. Hong, J.-Y. Park, F. Rotermund, D.-I. Yeom. Active control of all-fibre graphene devices with electrical gating. Nat. Commun., 6, 6861(2015).
[236] J. Bogusławski, Y. Wang, H. Xue, X. Yang, D. Mao, X. Gan, Z. Ren, J. Zhao, Q. Dai, G. Sobon, J. Sotor, Z. Sun. Graphene actively mode-locked lasers. Adv. Funct. Mater., 28, 1801539(2018).
[237] K. Chen, X. Zhou, X. Cheng, R. Qiao, Y. Cheng, C. Liu, Y. Xie, W. Yu, F. Yao, Z. Sun, F. Wang, K. Liu, Z. Liu. Graphene photonic crystal fibre with strong and tunable light–matter interaction. Nat. Photon., 13, 754(2019).
[238] S. Duval, M. Bernier, V. Fortin, J. Genest, M. Piché, R. Vallée. Femtosecond fiber lasers reach the mid-infrared. Optica, 2, 623(2015).
[239] T. Hu, S. D. Jackson, D. D. Hudson. Ultrafast pulses from a mid-infrared fiber laser. Opt. Lett., 40, 4226(2015).
[240] C. Zhu, F. Wang, Y. Meng, X. Yuan, F. Xiu, H. Luo, Y. Wang, J. Li, X. Lv, L. He, Y. Xu, J. Liu, C. Zhang, Y. Shi, R. Zhang, S. Zhu. A robust and tuneable mid-infrared optical switch enabled by bulk Dirac fermions. Nat. Commun., 8, 14111(2017).
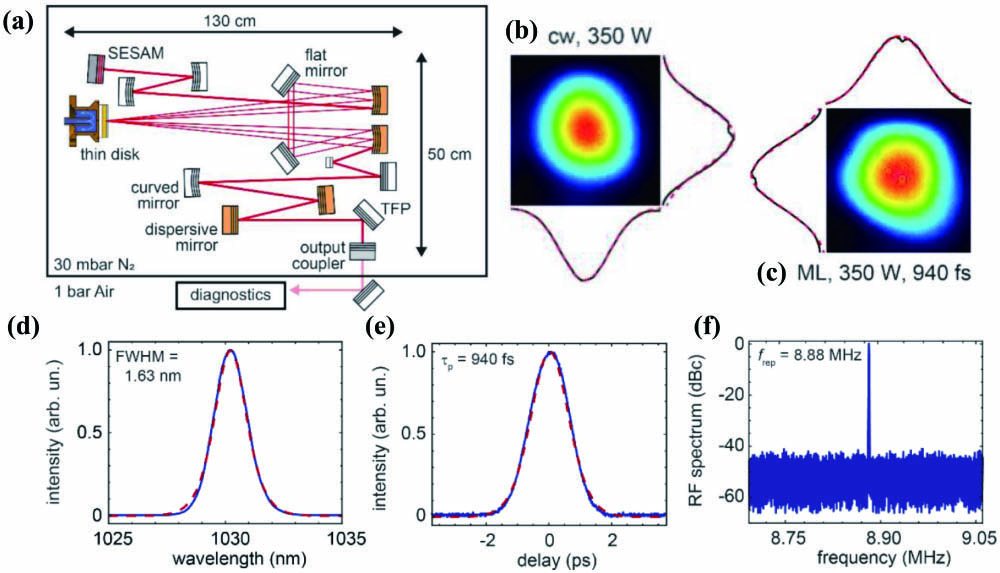
Set citation alerts for the article
Please enter your email address