Abstract
A serial line scan microscopic imaging system with 1 GHz scan rate is proposed and demonstrated. This method is based on optical time-stretch in dispersive fiber to realize superfast scan imaging. Furthermore, a wavelength division technique is utilized to overcome the trade-off between high frame rate and spatial resolution caused by dispersion-induced pulse overlap. Every single frame is carved into two channels by optical filters and is detected in different wavelength bands separately. Then, both channels are combined to reconstruct the whole frame. By this method, an imaging system with spatial resolution of 28 μm at line scan rate of 1 GHz with chromatic dispersion of 1377 ps∕nm is realized. It has the potential to capture fast, nonrepetitive transient phenomena with a timescale of less than one nanosecond.1. INTRODUCTION
In the domain of optical microscopic imaging technology, most of the current research aims to break the diffraction limit of spatial resolution [1,2]. However, enhancing the temporal resolution (i.e., frame rate) without sacrificing the detection sensitivity has been an imperative and challenging task, particularly in those applications where ultrafast measurements are essential, such as biomedical diagnostics [3], flow cytometry, and microfluidics [4]. For conventional imaging technologies, there have been the fundamental limitations that appear in standard charge-coupled devices (CCDs) [5] and complementary metal oxide semiconductors (i.e., a trade-off between high sensitivity and frame rate), which impedes the unraveling of the high-speed dynamical phenomena. In scientific research, ultrafast single-shot diffraction imaging with X rays [6] is proposed, but with the CCD as the detection device, and it only can capture repetitive events. Combining the use of a streak camera with repeated laser pulses can simulate a movie with a frame rate of one trillion frames per second [7], but this technique is only suitable for capturing an event that can be recreated exactly the same way multiple times. Recently, serial time-encoded amplified microscopy (STEAM) is demonstrated as a novel optical imaging technology that can achieve high frame rate () in real time [8–13]. STEAM is an optical method that uses a combination of spatially and temporally dispersive elements with a broadband mode-locked laser to achieve ultrafast single pixel imaging [14–20]. However, such an imaging system with the frame rate of megahertz only can capture transient phenomena with time scale more than nanoseconds. By increasing the repetition rate of the pulse laser up to gigahertz, we have achieved a line scan microscopic imaging system with 1 GHz scan rate [21]. To ensure adjacent image-encoded pulses with no temporal overlap, the largest dispersion is limited by the repetition rate of the pulse laser, and the final resolution of this imaging system with gigahertz frame rate will be restricted by such small dispersion. As we have reported, a resolution of 35 μm is achieved with the dispersion of . Continually increasing the dispersion will make the adjacent pulses overlap, and these pulses can’t be directly detected via digital sampling oscilloscope. It seems that there has been a trade-off between resolution and frame rate. For detecting smaller objects, this finite dispersion will influence the quality of images. In photonic time stretch, a continuous-time, large-bandwidth, time-stretched signal is segmented and interleaved into multiple parallel channels using virtual time gating (VTG), which makes every channel with no temporal overlap [22,23].
In this work, we propose and demonstrate a superfast serial wavelength-division microscopic imaging system with line scan rate of record 1 GHz. In order to achieve higher spatial resolution, we increase the group velocity dispersion (GVD) of fiber. The adjacent pulses have overlap in the temporal domain. Every image-encoded pulse is carved into two channels by wavelength division filters as VTG does, so the image can be reconstructed through these two channels. Such a superfast line scan imaging system has a field of view of 250 μm and spatial resolution of 28 μm. Based on this principle, we break the trade-off between large dispersion (limiting the spatial resolution) and high scan rate. As far as we know, this is the first time that the temporal resolution of real-time line scan imaging reaches 1 ns with the wavelength division technique. With a 1 GHz scan rate, this technique has potential to unlock the world of subnanosecond imaging.
2. PRINCIPLE AND EXPERIMENT
Our ultrafast imaging experimental setup is shown in Fig. 1. The 10 GHz ultrashort pulse laser (with center wavelength 1549.2 nm, 3 dB bandwidth nearly 1.2 nm, and pulse width 2 ps) is pulse-picked to reduce the repetition rate to 1 GHz using a gated Mach–Zehnder amplitude modulator. The spectral and temporal characteristics of the output pulse are shown in Fig. 1 (inset). The modulated pulses are amplified up to 26 dBm by a high-power Erbium-doped fiber amplifier. Then the pulses enter a free space optical link for imaging. The pulses are dispersed with a diffraction grating () to realize wavelength-to-space mapping. The one-dimensional (1D) space rainbow line focused by a spherical lens (focal length of 100 mm) is incident onto the sample. The rainbow pulses encoded with the information of the sample are reflected back and directed via an optical circulator towards a section of dispersion-compensating fiber (DCF, with dispersion of ) to realize the wavelength-to-time mapping. Different from our previous report [18], every pulse width after dispersion is more than 1 ns, which indicates that adjacent pulses have temporal overlap. This overlapped waveform cannot be detected directly, which would lose the original sample information. To solve this problem, at the receiver end, the temporal signal is equally split into two channels by different wavelength-band filters. The fundamental principle based on wavelength division technique is shown in Fig. 2. Here, the dispersed pulses are linearly chirped and adjacent pulses have overlap in the time domain. The temporal width of an entire pulse is determined as where is the spectral bandwidth of the optical pulse, is the GVD, and is the temporal width of an entire pulse. In this work, the relationship between the period of the pulse laser and temporal width of a pulse abides with the equation Here, is the period of the pulse laser. But different pulse contains different wavelength bands in the overlapped part. The overlapped part is carved into two parts with different wavelength bands. The temporal width of every half pulse out from each channel is less than the period of pulse laser, which demonstrates that there is no temporal overlap anymore for each channel. Finally, the signal of both channels with no temporal overlap is detected by high-speed photodetectors with the detection bandwidth of 10 GHz, and is digitized in real time by a high-speed oscilloscope with the sampling rate of . By moving the sample with the stepper motor in the orthogonal direction, the 1D spectral shower can be used to interrogate and line-scan the sample to acquire the two-dimensional (2D) image.
Sign up for Photonics Research TOC. Get the latest issue of Photonics Research delivered right to you!Sign up now
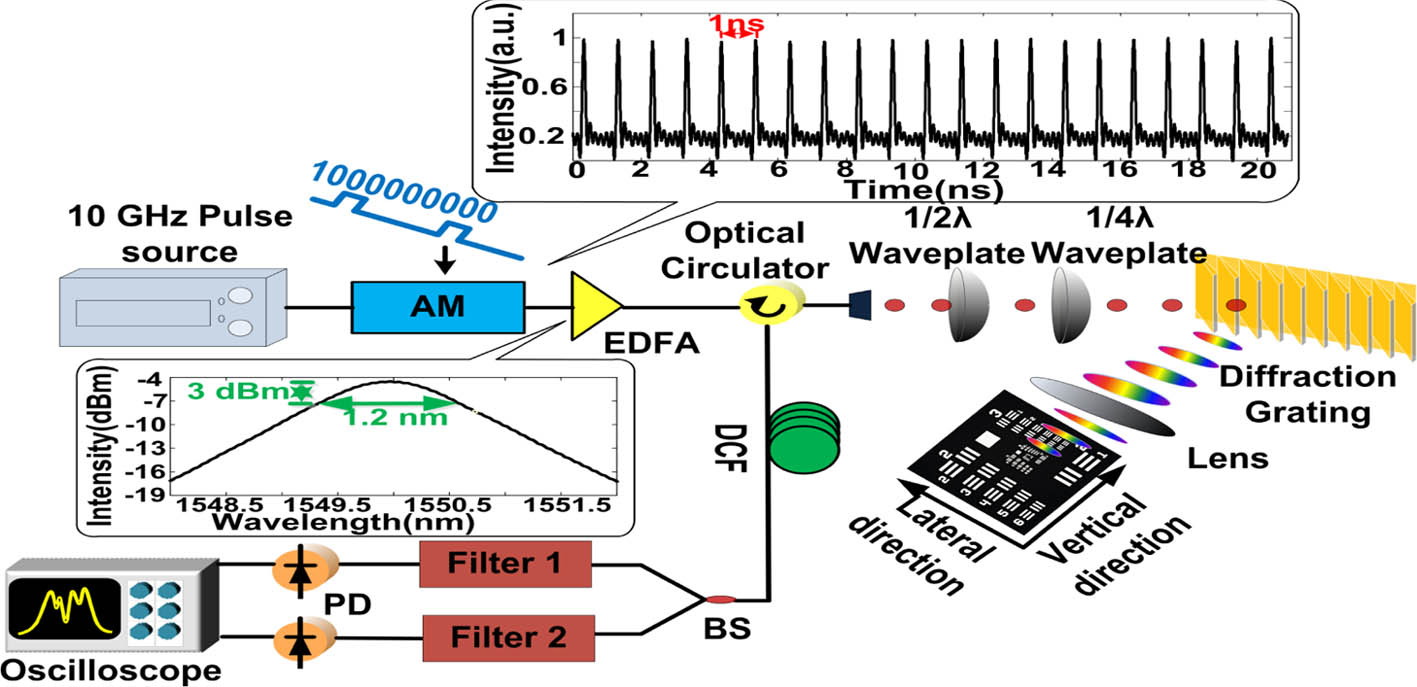
Figure 1.Experimental setup of the superfast serial wavelength-division line scan imaging system with 1 GHz. AM, amplitude modulator; EDFA, erbium-doped fiber amplifier; BS, beam splitter; PD, photodetector. Inset shows the temporal profile and spectrum of the amplitude-modulated 1 GHz pulse.
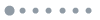
Figure 2.Principle of this wavelength-division imaging system. Inset shows the wavelength-to-time mapping for a whole pulse and each channel’s information after filters for a single pulse. Here, T represents the period of the mode-locked pulse laser, Z is the temporal width of the dispersed pulse, B is the spectral bandwidth, D is the GVD, and W is the width of pulse filtered from each channel.
3. RESULTS AND DISCUSSION
To demonstrate this microscopic imaging system performance, we take a test by imaging the USAF-1951 standard resolution target (group 4 element 2 with line width of 28 μm). Figure 3 shows the single-shot temporal waveform filtered from two channels. The pass bandwidth of filter 1 is from 1549 to 1549.75 nm, and that for filter 2 is from 1549.75 to 1550.5 nm. The filters are designed to be rectangle-shaped to decrease the distortion of spectrum, as shown in Fig. 3(b). Figure 3(c) shows the single-shot waveform of channel 1 and channel 2 captured from the red line in Fig. 3(a). Here, the size of the red line is . The combined temporal waveform resembles the spectral shape encoded with image [as shown in Figs. 3(b) and 3(c)], which demonstrates the wavelength-to-time mapping. Furthermore, as shown from Fig. 4, both channels after filters still work at 1 GHz scan rate but with smaller optical bandwidth. The 2D image achieved without wavelength division is shown in Fig. 5(a), which shows the blurred information due to temporal overlap. By using optical filters, the 2D images captured from two channels are illustrated as in Fig. 5(b). It can be seen that the information of the target is correctly recovered. It should be clear that filters have an intermediate spectral range between transmission and reflection spectra, which results in the loss of spectra in the intermediate range (corresponding to adjacent edge of two segments). In Fig. 5(b), some sort of compensation is required to fill this gap by strong digital filters.
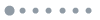
Figure 3.(a) Image of group 4 of a resolution target (USAF-1951), (b) spectrum captured from the red line in (a) measured by an optical spectrum analyzer, and (c) integrated single-shot temporal waveform captured from the red line in (a).
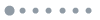
Figure 4.(a) Temporal waveform encoded with image captured from one channel by filter 1. (b) Waveform captured from the other channel by filter 2. The figure inset shows single-shot frame information. Both channels have no temporal overlap.
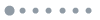
Figure 5.(a) 2D image acquired without wavelength-division and (b) 2D images acquired from two channels after optical filters.
Furthermore, for comparison, the 10 GHz mode-locked pulse source is pulse-picked to reduce the repetition rate to 100 MHz. With the GVD of , the temporal width of the pulse is less than the pulse period (10 ns). The sample information can be reconstructed directly without wavelength division. Here, the reconstructed image with line scan rate of 1 GHz is compared to the picture with line scan rate of 100 MHz, which is shown in Fig. 6. From Figs. 6(a) and 6(b), based on this wavelength division technique, the scan rate is improved without reducing the quality of image. This proof-of-principle demonstration validates the feasibility of wavelength-division 1 GHz imaging.
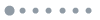
Figure 6.(a) Reconstructed 2D image with 100 MHz scan rate and (b) reconstructed 2D image with 1 GHz scan rate. It consists of two channels, which can be discerned from the boundary.
The spatial resolution of such a superfast imaging system in the scan direction, unlike conventional optical imaging, depends on three limitations: (1) the diffraction-limited optical link; (2) the spectral resolution of the DCF; (3) the temporal resolution of the optical detection system (i.e., the photodetector and digitizer) [24,25]. In our experiment, limitation (1) is defined by the grating and lens, which is 25 μm. With the bandwidth of 10 GHz (limited by photodetector), limitation (3) is . For limitation (2), in our previous experiment [21], to maintain no temporal overlap between adjacent pulses, the spatial resolution of 35 μm is achieved with the chromatic dispersion of . Here, the GVD is increased to and the resolution of limitation (2) is 28 μm. In the vertical direction, the sample is controlled by a step motor, and the distance of every step is 10 μm, which indicates the spatial resolution in the orthogonal direction. From Ref. [24], spatial resolution is proportional to dispersion amount. Dispersion is relative on line-scan rate determined by repetition rate of the mode-locked laser source. In our system, large dispersion makes adjacent pulses overlap. Wavelength division is used to avoid this overlap, and higher spatial resolution is achieved with respect to our previous report.
Generally, we take a deep consideration for this method. For a certain repetition rate of pulse laser source, larger dispersion (occupying more time scale with following pulses) can obtain higher spatial resolution but more optical filters are needed [as shown in Fig. 7(a)]. The number of filters is determined as where is the spectrum bandwidth of optical pulse, is the GVD, and is the period of pulse. With the parameter in our experiment, the number of filters is 2 [Fig. 7(b)]. Every single frame is recovered by two channels synchronously and combined together to get a whole image. On the other hand, for achieving higher scan rate, we also need more optical filters. By carving the single frame into multiple bands, we believe that the image can be reconstructed at much higher frame rate. With more filters and photodetectors, the weakness is that entire system becomes less compact compared with STEAM.
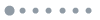
Figure 7.(a) Relationship diagram between T, B, D, and N, where T is the period of pulse source, B is the optical bandwidth, D is the GVD, and N is number of filters. (b) Constructed image with 1 GHz line scan rate; the 2D image is acquired with wavelength-division.
4. CONCLUSION
Temporal overlap between adjacent pulses limits the largest GVD in the time-stretch imaging system, which also limits the spatial resolution. Here, we report a novel wavelength-division line scan microscopic imaging system. This system releases the limitation between GVD and scan rate. A record 1 GHz real-time line scan microscopic imaging system is achieved with a field of view of 250 μm and spatial resolution of 28 μm. Through carving the temporal overlapping waveform by optical filters, each channel can be directly digitized in real-time. By moving the sample in the vertical direction, the 2D image is obtained. Compared with the previous method reported in Ref. [21], both cases work at scan rate of 1 GHz but with different spatial resolution (due to different dispersion amount). Here, larger dispersion improves the spatial resolution. In this demonstration, although the system works at the 1D condition, it is easily extended to 2D systems by replacing the 1D diffraction grating with a pair of orthogonally oriented spatial dispersers. We strongly believe that further development of the proposed higher frame rate imaging will be possible, based on the high repetition rate ultrashort pulse laser source and wavelength division technique. This ultra-high-speed imaging technique has potential to capture nonrepetitive transient phenomena of less than nanosecond duration.
References
[1] R. Zhang, Y. Zhang, Z. C. Dong, S. Jiang, C. Zhang, L. G. Chen, L. Zhang, Y. Liao, J. Aizpurua, Y. Luo, J. L. Yang, J. G. Hou. Chemical mapping of a single molecule by plasmon-enhanced Raman scattering. Nature, 498, 82-86(2013).
[2] D. G. de Oteyza, P. Gorman, Y. C. Chen, S. Wickenburg, A. Riss, D. J. Mowbray, G. Etkin, Z. Pedramrazi, H. Z. Tsai, A. Rubio, M. F. Crommie, F. R. Fischer. Direct imaging of covalent bond structure in single-molecule chemical reactions. Science, 340, 1434-1437(2013).
[3] K. Ohki, S. Chung, Y. H. Ch’ng, P. Kara, R. C. Reid. Functional imaging with cellular resolution reveals precise micro-architecture in visual cortex. Nature, 433, 597-603(2005).
[4] J. V. Watson. Introduction to Flow Cytometry(2004).
[5] T. G. Etoh, C. V. Le, Y. Hashishin, N. Otsuka, K. Takehara, H. Ohtake, T. Hayashida, H. Maruyama. Evolution of ultra-high-speed CCD imagers. Plasma Fusion Res., 2, S1021(2007).
[6] A. Barty, S. Boutet, M. J. Bogan, S. H. Riege, S. Marchesini, K. S. Tinten, N. Stojanovic, R. Tobey, H. Ehrke, A. Cavalleri, S. Düsterer, M. Frank, S. Bajt, B. W. Woods, M. M. Seibert, J. Hajdu, R. Treusch, H. N. Chapman. Ultrafast single-shot diffraction imaging of nanoscale dynamics. Nat. Photonics, 2, 415-419(2008).
[7] A. Velten, E. Lawson, A. Bardaqjy, M. Bawendi, R. Raskar. Slow art with a trillion frames per second camera. ACM SIGGRAPH 2011 Talks, 44(2011).
[8] K. Goda, K. K. Tsia, B. Jalali. Amplified dispersive Fourier-transform imaging for ultrafast displacement sensing and barcode reading. Appl. Phys. Lett., 93, 131109(2008).
[9] F. Qian, Q. Song, E. K. Tien, S. K. Kalyoncu, O. Boyraz. Real-time optical imaging and tracking of micron-sized particles. Opt. Commun., 282, 4672-4675(2009).
[10] K. Goda, K. K. Tsia, B. Jalali. Serial time-encoded amplified imaging for real-time observation of fast dynamic phenomena. Nature, 458, 1145-1149(2009).
[11] C. Zhang, Y. Qiu, R. Zhu, K. K. Wong, K. K. Tsia. Serial time-encoded amplified microscopy (STEAM) based on a stabilized picosecond supercontinuum source. Opt. Express, 19, 15810-15816(2011).
[12] T. T. Wong, A. K. Lau, K. K. Wong, K. K. Tsia. Optical time-stretch confocal microscopy at 1 μm. Opt. Lett., 37, 3330-3332(2012).
[13] Y. Qiu, J. Xu, K. K. Wong, K. K. Tsia. Exploiting few mode-fibers for optical time-stretch confocal microscopy in the short near-infrared window. Opt. Express, 20, 24115-24123(2012).
[14] A. M. Fard, S. Gupta, B. Jalali. Photonic time-stretch digitizer and its extension to real-time spectroscopy and imaging. Laser Photon. Rev., 7, 207-263(2013).
[15] K. Goda, A. Mahjoubfar, C. Wang, A. Fard, J. Adam, D. R. Gossett, A. Ayazi, E. Sollier, O. Malik, E. Chen, Y. Liu, R. Brown, N. Sarkhosh, D. D. Carlo, B. Jalali. Hybrid dispersion laser scanner. Sci. Rep., 2, 445(2012).
[16] K. Goda, A. Ayazi, D. R. Gossett, J. Sadasivam, C. K. Lonappan, E. Sollier, A. M. Fard, S. C. Hur, J. Adam, C. Murray, C. Wang, N. Brackbill, D. D. Carlo, B. Jalali. High-throughput single-microparticle imaging flow analyzer. Proc. Natl. Acad. Sci. USA, 109, 11630-11635(2012).
[17] K. Goda, B. Jalali. Dispersive Fourier transformation for fast continuous single-shot measurements. Nat. Photonics, 7, 102-112(2013).
[18] F. Xing, H. Chen, M. Chen, S. Yang, S. Xie. Simple approach for fast real-time line scan microscopic imaging. Appl. Opt., 52, 7049-7053(2013).
[19] H. Chen, C. Wang, A. Yazaki, C. Kim, K. Goda, B. Jalali. Ultrafast web inspection with hybrid dispersion laser scanner. Appl. Opt., 52, 4072-4076(2013).
[20] T. T. Wong, A. K. Lau, K. K. Ho, M. Y. Tang, J. D. Robles, X. Wei, A. C. Chan, A. H. Tang, E. Y. Lam, K. K. Wong, G. C. Chan, H. C. Shum, K. K. Tsia. Asymmetric-detection time-stretch optical microscopy (ATOM) for ultrafast high-contrast cellular imaging in flow. Sci. Rep., 4, 3656(2014).
[21] F. Xing, H. Chen, M. Chen, S. Yang, H. Yu, S. Xie. World’s fastest real-time line scan microscopic imaging system with 1 GHz frame rate. Lasers and Electro-Optics Pacific Rim (CLEO-PR), 1-2(2013).
[22] Y. Han, B. Jalali. Continuous-time time-stretched analog-to-digital converter array implemented using virtual time gating. IEEE Trans. Circuits Syst. I, 52, 1502-1507(2005).
[23] J. H. Wong, H. Q. Lam, R. M. Li, K. E. K. Lee, V. Wong, P. H. Lim, S. Aditya, P. P. Shum, S. H. Xu, K. Wu, C. Ouyang. Photonic time-stretched analog-to-digital converter amenable to continuous-time operation based on polarization modulation with balanced detection scheme. J. Lightwave Technol., 29, 3099-3106(2011).
[24] K. K. Tsia, K. Goda, D. Capewell, B. Jalali. Performance of serial time-encoded amplified microscopy. Opt. Express, 18, 10016-10028(2010).
[25] K. Goda, D. R. Solli, K. K. Tsia, B. Jalali. Theory of amplified dispersive Fourier transformation. Phys. Rev. A, 80, 043821(2009).