Abstract
The experimental investigation of mode distortion induced by stimulated Raman scattering (SRS) in a high-power fiber amplifier, which includes the evolutions of optical spectra, spatial beam profiles, and time-frequency characteristics, has been carried out in detail. Temporal-frequency characteristics have been studied for the first time, to the best of our knowledge, by using a low-speed camera and high-speed photodiode traces, which revealed that temporal-frequency characteristics of SRS-induced mode distortion are different from traditional dynamic mode instability (MI). The experimental results show that the output beam profile remains stable before the mode distortion occurs and fluctuates obviously after the onset of SRS-induced MI but on a time scale of seconds, which is much lower than that of Yb-gain-induced MI featuring millisecond-level beam profile fluctuation. It also shows that the mode distortion became measurable in company with the onset of inter-mode four-wave mixing (IM-FWM) when the ratio of Raman light reaches 3%; further, the beam quality factor degrades gradually from 1.4 to 2.1 as the ratio of Raman light increases. The mode distortion is accompanied by an obvious temperature increase of the output passive fiber, which further confirms that the mode distortion originates from SRS. The cause of the mode distortion induced by SRS has been explained in the context of core-pumped SRS effect, and the investigation on the accompanying IM-FWM effect indicates that the main content of the SRS-induced high-order mode is the LP21 mode.1. INTRODUCTION
Due to high beam quality, easy thermal management, high robustness, and compact size, the fiber laser is becoming one of the most promising laser sources for high-power scaling with excellent beam quality. In recent years, fiber lasers have demonstrated rapid evolution in terms of output power and are now widely used in industrial materials processing, medical sciences, spectroscopy, and so forth [1–4]. However, due to high intensity in the fiber core, nonlinear effects can menace the scaling of output power of fiber lasers, especially stimulated Raman scattering (SRS) and mode instability (MI) for industrial high-power continuous-wave (CW) fiber lasers [5–9]. The SRS effect will transfer the desired signal power to a longer wavelength nonlinearly when the output power exceeds the SRS threshold [10–13], which reduces the effective signal power content. In order to suppress the SRS effect, large-mode area (LMA) fibers are commonly employed in high-power fiber laser systems. The LMA fibers can support a few transverse modes; thus, MI will occur when the output power reaches a certain threshold, which will degrade the beam quality and pointing stability of the fiber laser systems [7]. Over the past decade, there have been many theoretical and experimental reports on the MI phenomenon [14–21]; further, the SRS was thought to be independent of MI until researchers found SRS-induced MI in 2017 [22]. In 2016, Naderi et al. predicted that the Raman conversion could cause MI in Raman fiber lasers [23], which was different from the experimental phenomenon of SRS-induced MI [24]. It is reported that SRS-induced MI happens in signal light instead of Stokes light [24]. However, according to the theoretical results, SRS-induced MI should happen in Stokes light [23]. Then, another explanation of the underlying physical mechanism of SRS-induced MI was introduced [25], which attributed the phenomenon to the intermode four-wave mixing (IM-FWM) but also cannot explain why SRS-induced MI happens in a signal laser. In addition, one can find the temporal characteristics of SRS-induced MI are different from those of traditional MI by comparing the results in Ref. [16] and [22], which have not been analyzed in detail.
In this paper, the experimental study on SRS-induced mode distortion has been carried out in a counter-pumped all-fiberized amplifier; further, the temporal characteristics of SRS-induced mode distortion have been studied with the help of the Fourier analysis, which is proved to be a useful method to investigate the mechanism and characteristics of SRS-induced mode distortion phenomenon. The experiment results have been discussed, and the mode distortion is considered as the result of fiber core-pumped SRS effect.
2. EXPERIMENT SETUP
The all-fiber laser system based on a master oscillator power amplifier (MOPA) configuration is established, as shown in Fig. 1. The system is composed of a master oscillator and a power amplifier. The oscillator consists of a high reflector (HR), a 10/130 Yb-doped fiber (YDF), and an output coupler (OC). The central wavelength of gratings is 1064 nm, and their reflectivity is 99.9% and 10%, respectively. In order to study SRS-induced MI, we intentionally use a narrow linewidth oscillator to trigger an SRS effect [26–28]; further, the linewidths of HR and OC are 2 and 0.1 nm, respectively. A 50 W 976 nm laser diode (LD) is used to pump the cavity via a combiner, which can deliver a maximal output power of 30 W. Then, the seed laser is coupled to the main amplifier stage through the multifunctional mode field adapter, which is combined with a cladding power stripper (CPS). The amplifier stage is made up of a counter signal/pump combiner, a piece of LMA YDF with core diameter 26 μm and cladding diameter of 400 μm, two CPSs, and an output quartz block holder (QBH). The pump absorption coefficient of the LMA YDF is at 915 nm. The main amplifier stage is pumped by six pump modules via a signal/pump combiner. Each pump module is made up of a pump combiner with 150 W LD connected to each arm; in addition, the central wavelength of LD is 915 nm. The pumping schemes were chosen to increase the threshold of Yb-gain-induced MI [29,30] and avoid the influence of Yb-gain-induced MI on SRS-induced MI [29,30]. The CPSs are utilized to remove the residue pump power and the unwanted cladding signal light. To strip the high-order mode (HOM) and obtain good beam quality, the active fiber is coiled, and the fiber systems were water-cooled on a heat sink for efficient thermal management.
Sign up for Photonics Research TOC. Get the latest issue of Photonics Research delivered right to you!Sign up now
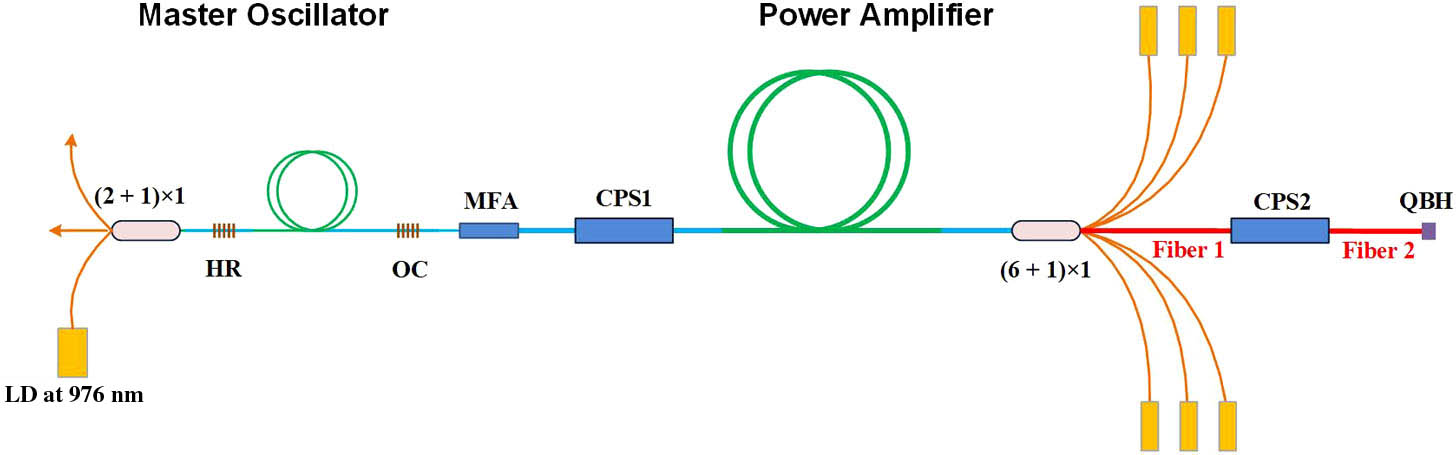
Figure 1.Experiment setup of the laser system.
3. EXPERIMENTAL RESULTS
The seed is injected into the main amplifier, and the output power of the amplifier increases linearly with the pump power, as shown in Fig. 2(a). When the injected pump power is 2.60 kW, the output power is 1.91 kW, and optical-to-optical efficiency is 72.7%. The spectra at different output powers are presented in Fig. 2(b). With the scaling of output power, the spectrum linewidth of the laser broadens, which is due to self-phase modulation and the four-wave mixing effect in the amplifier. Furthermore, the light ranging from 1100 to 1140 nm is caused by the SRS effect, while the light rising around 1032 nm is due to the IM-FWM [31]. When the output power is 1.91 kW, the SRS signal-to-noise ratio is 18 dB, and the ratio of the SRS light power to total output power is 13.87%, which is calculated through the spectral integration from 1100 to 1140 nm.
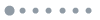
Figure 2.(a) Output power of the laser as a function of pump power. (b) Spectra at different output powers.
The Raman ratio and IM-FWM ratio at different output powers are illustrated in Fig. 3. One can see that both the ratios of Raman and IM-FWM increase obviously when the output power exceeds 1560 W. The beam quality of the laser is measured, and the for the laser at several output powers is illustrated in Fig. 3(a). One can see that, when the output power is lower than 1560 W, the ratio of SRS is less than about 3%, and the beam quality degrades slightly as the output power increases. When the output power is 1560 W, the beam quality of the laser is , . The mode degradation is because the injecting seed laser is not strictly single mode, and the gain of the HOM is higher in the amplifier, which results in the increasing of the fraction of HOM with the scaling of output power [32]. After the ratio of SRS exceeds 3%, the beam quality degrades sharply as the output power of signal laser increases, and the beam quality is degraded to , at 1910 W. Therefore, the obvious SRS effect will cause mode degradation. The onset power of mode degradation and IM-FWM is nearly the same, which is because the IM-FWM is caused by the nonlinear interaction between the fundamental mode and HOM; thus, the HOM induced by SRS will stimulate the IM-FWM simultaneously.
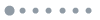
Figure 3.(a) Beam quality and the ratio of Raman Stokes light. (b) Ratio of IM-FWM light of the laser at different output powers.
With the scaling of output power after the onset of SRS, the temperature of the passive fiber (fiber 1 and fiber 2, marked red in Fig. 1) will increase due to the quantum defect during the Raman conversion process. The highest temperature, which is located in fiber 2 near the QBH, has been measured in experiments, as shown in Fig. 4. One can see that, when the ratio of SRS is less than 2%, the heat load in the passive fiber can be dissipated effectively by convection; further, the temperature of the passive fiber will maintain the room temperature. When the ratio of the SRS increases beyond 2%, the heat load increases beyond the capability of the convection, and the temperature of the fiber increases obviously. The results confirm that the mode degradation of the signal laser is caused by the onset of SRS but needs to reach a certain level of Raman power. The aforementioned results agree with the discussion in Ref. [33], where the SRS is weaker, and no SRS-induced mode degradation has been observed.
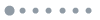
Figure 4.Temperature of passive fiber of the laser at different output powers.
We use a camera to study the mode distortion, spatially and dynamically. Figure 5 shows the near-field intensity from the camera at different output powers. Visualization 1 and Visualization 2 show the recorded videos of the near-field beam-profile evolution within a time period of 10 s at 1560 W (lower than MI threshold) and 1910 W (higher than MI threshold) output power, respectively. It shows that the beam profiles are stable when the output power is lower than MI threshold at 1560 W but fluctuate when the MI occurs at 1910 W.
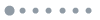
Figure 5.Near-field intensity distributions captured from camera at (a) 1560 W (Visualization 1) and (b) 1910 W (Visualization 2).
To study the temporal dynamics in detail, we use a simple photodiode (PD) to measure the transversal beam profile, which provides important characteristics of the temporal dynamics such as their temporal periodicity or noise. A PD with a hole of 1.0 mm diameter and bandwidth of 350 MHz is placed in the center of the collimated beam to detect the temporal characteristics of SRS-induced mode distortion. The bandwidth of oscilloscope used in the experiment is 500 MHz. The time traces at six different output power levels are shown in Fig. 6. Figure 6(a) depicts the time traces for a time period of 50 s with 200 kSa/s sampling rate; Fig. 6(b) illustrates the time traces for a time period of 100 μs with 200 MSa/s sampling rate, which expresses the power fluctuation at the Hz and MHz levels, respectively. From Fig. 6(a), one can see that, with the scaling of output power, the time trace is stable before the onset of mode distortion, and the time trace fluctuates obviously when SRS-induced mode distortion occurs. However, the time trace fluctuates at a much slower speed, which indicates the slow-speed energy conversion of the mode power; further, the result rules out the thermal origin of SRS-induced MI [16]. From Fig. 6(b), one can see that the time trace always keeps stable with the scaling of output power, which indicates that the MI is not caused by the backward SRS-induced seed’s inversion [34]. In Refs. [35,36], SRS-induced MI has not been observed even when the Raman Stokes light is only about 10 dB below the intensity of signal light, which is because the bend loss of HOM is high and the variation of HOM fraction is ignorable. In Ref. [36], the fraction of HOM is high, as indicated by its beam quality, so the SRS results in obvious mode degradation.
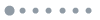
Figure 6.Time traces of the laser with (a) 50 s period and (b) 100 μs period at different output powers.
Furthermore, we applied Fourier analysis on the time traces to calculate the corresponding Fourier spectrum (FS) to simplify the investigation on the periodicity of the time traces. The FSs of the time traces with 50 s period at different output power levels are shown in Fig. 7. One can see that, with the onset of SRS-induced MI, the frequency component lower than 5 Hz increases; at higher frequencies, the noise remains at the dark noise level and does not show additional noise features. The FS results indicate that the time traces in the region exhibit a time fluctuation at the several-second level and that the Yb-gain-induced MI has not been triggered.
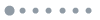
Figure 7.FS of low-frequency time traces with 50 s period.
Figure 8 shows the relative intensity as a function of output power. This relative intensity is defined as , where is the power density at the frequency of . Below the threshold, in the range from 0 to 200 Hz is nearly the same as that at 200–400 Hz, which results in the fact that is around zero. After mode distortion happens, the frequency component shows up in the range of 0–200 Hz, which results in the fact that the value of increases sharply and indicates the onset of MI [37].
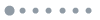
Figure 8.Character factor as a function of output laser power.
One effective method to suppress HOM is to introduce mode-specific bend loss [38,39]. In order to suppress SRS-induced mode distortion, the passive fiber (fiber 1) before CPS2 is bent with about an 8 cm bend diameter and about an 0.3 m bend length. The results are shown in Fig. 9. Figure 9(a) shows the output power versus pump power; with 2.60 kW pump power, the output power is 1.85 kW, and optical-to-optical efficiency is 70.8%. After the fiber is bent, the efficiency decreases, which is because the HOM is leaked to the fiber cladding in the bent fiber and is stripped by CPS. The beam quality versus output power is shown in Fig. 9(b). SRS-induced MI threshold is about 1520 W; further, compared with Fig. 3(a), one can conclude that the fiber bend has little impact on the threshold of SRS-induced mode distortion, which is in coincidence with the results in Ref. [22]. This is because the SRS effect starts at the beginning of the amplifier, and the SRS effect is already stimulated with the fraction of the HOM increasing at the end of the amplifier. The bend of the end part of the system can only strip the HOM instead of suppressing the HOM, which only results in reduction of efficiency.
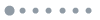
Figure 9.(a) Output power versus pump power. (b) Beam quality of the laser with bent passive fiber.
4. DISCUSSION
According to the experimental results, one can conclude that the cause of SRS-induced MI is different from Yb-gain-induced MI, and the experimental phenomenon is similar to that of a single-mode Raman fiber laser based on a multimode fiber [40]. One possible explanation of SRS-induced mode distortion is due to the core-pumped SRS effect. For the fiber laser, the output laser propagates in the fiber core, and the laser is the pump light of the SRS effect, so the SRS effect in a fiber amplifier is a core-pumped process. The signal laser is near fundamental mode, and the power distribution is Gaussian-shaped before the SRS occurs, so the power intensity near the fiber center is higher, which results in a stronger SRS effect in the center of the core and much lower SRS in the outer area of the core. Therefore, the result is that the light power distributing at the fiber center part decreases; then, the signal power distribution degrades from a Gaussian shape to super-Gaussian shape and then to a hollow shape, as shown in Fig. 10. One can see from the beam profiles that the HOM is mainly an LP21 mode. The temporal dynamics of SRS-induced mode distortion is due to the phase fluctuation between the fundamental mode and HOM caused by temperature variations, mechanical vibration, acoustic noise, seismic noise, etc., which cannot be avoided in a practical engineering environment and lies in a frequency range well below several hundred Hz in a relatively quiet laboratory environment [41–43].
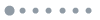
Figure 10.Near-field intensity distributions at several output powers.
Figure 3(b) shows that the IM-FWM effect will be stimulated after the onset of SRS-induced mode distortion, which is caused by the interaction between the fundamental mode and HOMs. In order to assess the main proportion of HOM, the frequency shifts caused by the IM-FWM effect between different modes are investigated. According to Ref. [44], the waveguide dispersion () and material dispersion () are considered to determine the phase-matching condition. In Fig. 11, we plot the curves of and versus the frequency shift, where calculation parameters are the same as in the experimental setup; further, the intersection of the curve of and is the phase-matching frequency shift of the IM-FWM process. For the case in which the signal light at the LP21 and LP01 modes produces the Stokes light at the LP21 and LP01 modes, the frequency shift of IM-FWM is 8.7 THz. For lasers operating at 1064 nm, the Stokes peak wavelength is 1097.88 nm with the anti-Stokes peak wavelength being 1032.15 nm, which is in coincidence with the spectrum in Fig. 2(b). Therefore, one can conclude that SRS-caused HOM is mainly the LP21 mode.
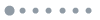
Figure 11.Phase-matching diagrams of the IM-FWM for the 26/400 fiber.
5. CONCLUSION
In conclusion, SRS-induced mode distortion is studied in an LMA step-index fiber in a counter-pumped all-fiberized amplifier. When the output power is scaled to 1560 W, the ratio of SRS is 2%, and SRS power begins to increase nonlinearly; then, the mode distortion occurs when the ratio of SRS reaches 3%, i.e., SRS-induced mode distortion. The beam quality factor of the fiber amplifier is measured, which is about 1.4 before the onset of mode distortion, and degrades after the onset of SRS-induced mode distortion and is about 2.1 at 1910 W. The output passive fiber temperature also begins to increase when the ratio of SRS exceeds 2%. The temporal dynamics of SRS-induced mode distortion is studied in detail; these dynamics are characterized via camera measurement and photodiode traces. The experimental results express that SRS-induced mode distortion is a slowly-varying process with beam profile fluctuation at the second level, which can be explained by the core-pumped SRS effect. With the onset of mode distortion, the IM-FWM effect will be stimulated simultaneously, and the investigation of IM-FWM indicates SRS-induced HOM is mainly in the LP21 mode.
References
[1] M. Zervas, C. Codemard. High power fiber lasers: a review. IEEE J. Sel. Top. Quantum Electron., 20, 219-241(2014).
[2] Z. Liu, X. Jin, R. Su, P. Ma, P. Zhou. Development status of high power fiber lasers and their coherent beam combination. Sci. China Inf. Sci., 62, 41301(2019).
[3] W. Shi, Q. Fang, X. Zhu, R. A. Norwood, N. Peyghambarian. Fiber lasers and their applications [invited]. Appl. Opt., 53, 6554-6568(2014).
[4] Y. Wang, G. Chen, J. Li. Development and prospect of high-power doped fibers. High Power Laser Sci. Eng., 6, e40(2018).
[5] Q. Fang, J. Li, W. Shi, Y. Qin, Y. Xu, X. Meng, R. A. Norwood, N. Peyghambarian. 5 kW near-diffraction-limited and 8 kW high-brightness monolithic continuous wave fiber lasers directly pumped by laser diodes. IEEE Photon. J., 9, 1506107(2017).
[6] F. Mäller, R. Krömer, C. Matzdorf, S. Nolte, M. Strecker, F. Stutzki, M. Plätner, V. Bock, T. Schreiber, A. Tünnermann. Comparison between bidirectional pumped Yb-doped all-fiber single-mode amplifier and oscillator setup up to a power level of 5 kW. Advanced Solid-State Lasers Congress, AM2A.3(2018).
[7] C. Jauregui, J. Limpert, A. Tünnermann. High-power fibre lasers. Nat. Photonics, 7, 861-867(2013).
[8] F. Mäller, R. Krömer, C. Matzdorf, M. Strecker, M. Plätner, F. Stutzki, T. Walbaum, T. Schreiber, R. Eberhardt, S. Nolte, A. Tünnermann. Multi-kW performance analysis of Yb-doped monolithic single-mode amplifier and oscillator setup. Proc. SPIE, 10897, 108970D(2019).
[9] H. Zhang, P. Zhou, H. Xiao, J. Leng, R. Tao, X. Wang, J. Xu, X. Xiaojun, Z. Liu. Toward high-power nonlinear fiber amplifier. High Power Laser Sci. Eng., 6, e51(2018).
[10] H. Zhang, H. Xiao, P. Zhou, X. Wang, X. Xu. High power Yb-Raman combined nonlinear fiber amplifier. Opt. Express, 22, 10248-10255(2014).
[11] L. Zhang, H. Jiang, S. Cui, Y. Feng. Integrated ytterbium-Raman fiber amplifier. Opt. Lett., 39, 1933-1936(2014).
[12] P. Ma, H. Zhang, L. Huang, X. Wang, P. Zhou, Z. Liu. Kilowatt-level near-diffraction-limited and linear-polarized ytterbium-Raman hybrid nonlinear amplifier based on polarization selection loss mechanism. Opt. Express, 23, 26499-26508(2015).
[13] Q. Xiao, P. Yan, D. Li, J. Sun, X. Wang, Y. Huang, M. Gong. Bidirectional pumped high power Raman fiber laser. Opt. Express, 24, 6758-6768(2016).
[14] A. V. Smith, J. J. Smith. Mode instability in high power fiber amplifiers. Opt. Express, 19, 10180-10192(2011).
[15] B. Ward, C. Robin, I. Dajani. Origin of thermal modal instabilities in large mode area fiber amplifiers. Opt. Express, 20, 11407-11422(2012).
[16] H.-J. Otto, F. Stutzki, F. Jansen, T. Eidam, C. Jauregui, J. Limpert, A. Tünnermann. Temporal dynamics of mode instabilities in high-power fiber lasers and amplifiers. Opt. Express, 20, 15710-15722(2012).
[17] K. Hejaz, A. Norouzey, R. Poozesh, A. Heidariazar, A. Roohforouz, R. R. Nasirabad, N. T. Jafari, A. H. Golshan, A. Babazadeh, M. Lafouti. Controlling mode instability in a 500 W ytterbium-doped fiber laser. Laser Phys., 24, 025102(2014).
[18] M. Kuznetsov, O. Vershinin, V. Tyrtyshnyy, O. Antipov. Low-threshold mode instability in Yb3+-doped few-mode fiber amplifiers. Opt. Express, 22, 29714-29725(2014).
[19] K. R. Hansen, J. Lægsgaard. Impact of gain saturation on the mode instability threshold in high-power fiber amplifiers. Opt. Express, 22, 11267-11278(2014).
[20] M. Zervas. Power scaling limits in high power fiber amplifiers due to transverse mode instability, thermal lensing, and fiber mechanical reliability. Proc. SPIE, 10512, 1051205(2018).
[21] R. Tao, X. Wang, P. Zhou. Comprehensive theoretical study of mode instability in high-power fiber lasers by employing a universal model and its implications. IEEE J. Sel. Top. Quantum Electron., 24, 0903319(2018).
[22] K. Hejaz, M. Shayganmanesh, R. Rezaei-Nasirabad, A. Roohforouz, S. Azizi, A. Abedinajafi, V. Vatani. Modal instability induced by stimulated Raman scattering in high-power Yb-doped fiber amplifiers. Opt. Lett., 42, 5274-5277(2017).
[23] S. Naderi, I. Dajani, J. Grosek, T. Madden. Theoretical and numerical treatment of modal instability in high-power core and cladding-pumped Raman fiber amplifiers. Opt. Express, 24, 16550-16565(2016).
[24] H. Lin, R. Tao, C. Li, B. Wang, C. Guo, Q. Shu, P. Zhao, L. Xu, J. Wang, F. Jing, Q. Chu. 3.7 kW monolithic narrow linewidth single mode fiber laser through simultaneously suppressing nonlinear effects and mode instability. Opt. Express, 27, 9716-9724(2019).
[25] W. Liu, P. Ma, C. Shi, P. Zhou, Z. Jiang. Theoretical analysis of the SRS-induced mode distortion in large-mode area fiber amplifiers. Opt. Express, 26, 15793-15803(2018).
[26] M. Hu, W. Ke, Y. Yang, M. Lei, K. Liu, X. Chen, C. Zhao, Y. Qi, B. He, X. Wang, J. Zhou. Low threshold Raman effect in high power narrowband fiber amplifier. Chin. Opt. Lett., 14, 011901(2016).
[27] A. Liem, E. Freier, C. Matzdorf, V. Reichel, T. Schreiber, R. Eberhardt, A. Tünnermann. Experimental analysis of the influence of the spectral width of out-coupling fiber Bragg gratings to the amount of stimulated Raman scattering in a cw kW fiber oscillator. Advanced Solid-State Lasers Congress, JTh2A.32(2013).
[28] W. Liu, P. Ma, H. Lv, J. Xu, P. Zhou, Z. Jiang. General analysis of SRS-limited high-power fiber lasers and design strategy. Opt. Express, 24, 26715-26721(2016).
[29] R. Tao, P. Ma, X. Wang, P. Zhou, Z. Liu. Mitigating of modal instabilities in linearly-polarized fiber amplifiers by shifting pump wavelength. J. Opt., 17, 045504(2015).
[30] R. Tao, P. Ma, X. Wang, P. Zhou, Z. Liu. Theoretical study of pump power distribution on modal instabilities in high power fiber amplifiers. Laser Phys. Lett., 14, 025002(2016).
[31] K. Shima, S. Ikoma, K. Uchiyama, Y. Takubo, M. Kashiwagi, D. Tanaka. 5-kW single stage all-fiber Yb-doped single-mode fiber laser for materials processing. Proc. SPIE, 10512, 45-50(2018).
[32] L. Huang, L. Kong, J. Leng, P. Zhou, S. Guo, X. Cheng. Impact of high-order-mode loss on high-power fiber amplifiers. J. Opt. Soc. Am. B, 33, 1030-1037(2016).
[33] R. Tao, H. Xiao, H. Zhang, J. Leng, X. Wang, P. Zhou, X. Xu. Dynamic characteristics of stimulated Raman scattering in high power fiber amplifiers in the presence of mode instabilities. Opt. Express, 26, 25098-25110(2018).
[34] V. Scarnera, F. Ghiringhelli, A. Malinowski, C. A. Codemard, M. K. Durkin, M. N. Zervas. Modal instabilities in high power fiber laser oscillators. Opt. Express, 27, 4386-4403(2019).
[35] C. Shi, R. T. Su, H. W. Zhang, B. L. Yang, X. L. Wang, P. Zhou, X. J. Xu, Q. S. Lu. Experimental study of output characteristics of bi-directional pumping high power fiber amplifier in different pumping schemes. IEEE Photon. J., 9, 1502910(2017).
[36] Z. Li, C. Li, Y. Liu, Q. Luo, H. Lin, Z. Huang, S. Xu, Z. Yang, J. Wang, F. Jing. Impact of stimulated Raman scattering on the transverse mode instability threshold. IEEE Photon. J., 10, 1502709(2018).
[37] R. Tao, P. Ma, X. Wang, P. Zhou, Z. Liu. Comparison of the threshold of thermal-induced mode instabilities in polarization-maintaining and non-polarization-maintaining active fibers. J. Opt., 18, 065501(2016).
[38] R. Tao, P. Ma, X. Wang, P. Zhou, Z. Liu. 1.3 kW monolithic linearly polarized single-mode master oscillator power amplifier and strategies for mitigating mode instabilities. Photon. Res., 3, 86-93(2015).
[39] M. Lei, Y. Qi, C. Liu, Y. Yang, Y. Zheng, J. Zhou. Mode controlling study on narrow-linewidth and high power all-fiber amplifier. Proc. SPIE, 9543, 95431L(2015).
[40] S. H. Baek, W. B. Roh. Single-mode Raman fiber laser based on a multimode fiber. Opt. Lett., 29, 153-155(2004).
[41] P. Ma, P. Zhou, Y. Ma, R. Su, Z. Liu. Coherent polarization beam combining of four high-power fiber amplifiers using single-frequency dithering technique. IEEE Photon. Technol. Lett., 24, 1024-1026(2012).
[42] Y. Ma, P. Zhou, X. Wang, H. Ma, X. Xu, L. Si, Z. Liu, Y. Zhao. Coherent beam combination with single frequency dithering technique. Opt. Lett., 35, 1308-1310(2010).
[43] D. C. Jones, C. D. Stacey, A. M. Scott. Phase stabilization of a large-mode-area ytterbium-doped fiber amplifier. Opt. Lett., 32, 466-468(2007).
[44] L. Yin, Z. Han, H. Shen, R. Zhu. Suppression of inter-modal four-wave mixing in high-power fiber lasers. Opt. Express, 26, 15804-15818(2018).