
- Photonics Research
- Vol. 9, Issue 10, 2037 (2021)
Abstract
1. INTRODUCTION
Due to the unique optical nature of lanthanide ions (
Recently, various combinations of dye-sensitized
In this work, we present the synthesis, emission properties, and a deep investigation of the photocycle of ultrasmall (
2. EXPERIMENT
A. Synthesis
Monodisperse
FITC-functionalized
B. Characterization
Powder X-ray diffraction (XRD) patterns were recorded on a Thermo Scientific ARL X’TRA diffractometer in the range of 20°–70°
C. Theoretical Calculations
The ground-state electronic structures of FITC as free dianion and coordinated to a
3. RESULTS AND DISCUSSION
The schematic energy level diagram of FITC and
Figure 1.Mechanism of ET in FITC sensitized
Figure 2.(a) TEM image and (b) powder XRD pattern of
The absorption and steady-state PL spectra in the Vis range of the free FITC in its dianionic form,
Figure 3.(a) Absorption spectra; (b) Vis emission spectra (
The change of the steady-state optical features of FITC when combined with the
Figure 4.(a) Optimized geometries and (b) MOs calculated by DFT methods at B3LYP/6-311 + G(d,p) level of theory (color codes: Ca, green; C, gray; O, red; N, purple; S, yellow; H, white). The orbitals are reported with a contour value of 0.050.
On the other hand, as previously mentioned, the change of the electronic properties of the dye moiety upon coordination, while leading to a significant variation of the molecular dipole moment, does not lead to a noticeable variation of the overall oscillator strength
The highly efficient ET from FITC to
Figure 5.(a) NIR emission spectra of
TA spectroscopy measurements have been carried out to analyze the excited state evolution of the dye molecules, which can shed light on the ET pathways of dye-sensitized lanthanide (Ln)-doped fluoride luminescent nanoparticles. Both radiative and nonradiative channels can be unravelled from TA maps by probing the differential absorbance
Figure 6.Two-dimensional (2D) TA (
The intensity of the TA features reaches a maximum within 1 ps and subsequently decreases as the time delays to 120 ps, with no significant changes in the spectral shape. The nearly instant signal rising in the entire probed range indicates that the TA responses of the three samples are all dominated by the excited singlet state
The kinetic trace for the
FITC-Excited Singlet Ultrafast Decay Time Constants and FITC to Er3+ Sensitization Efficiency Calculated through Eq. (
Sample | ||
---|---|---|
FITC | – | |
200 (10) | – | |
15 (2) | 92 |
Figure 7.(a) Spectral overlap of
The overall ET efficiency from the two populations of dyes in the “optimal” and “distorted” geometry can be estimated by considering the ratio of the fast and slow decay components in the TA decay trace of the reference
4. CONCLUSIONS
In conclusion, we have provided the first in-depth study of the structural, electronic, and photophysical properties of dye-sensitized
These results highlight that, to achieve highly efficient ET in dye-sensitized Ln-doped nanoparticles, dye molecules must be reasonably designed to have suitable excited singlet energy states and localized MOs (transition dipole moments) close to the surface of the nanoparticle. This proof of concept of dye-sensitized
Acknowledgment
Acknowledgment. We acknowledge critical and quantity of testing work supported by Beijing Zhongkebaice Technology Service Co., Ltd.
References
[1] J. Liu, A. M. Kaczmarek, R. V. Deun. Advances in tailoring luminescent rare-earth mixed inorganic materials. Chem. Soc. Rev., 47, 7225-7238(2018).
[2] J.-C. G. Bünzli, C. Piguet. Taking advantage of luminescent lanthanide ions. Chem. Soc. Rev., 34, 1048-1077(2005).
[3] F. Artizzu, F. Quochi, L. Marchiò, C. Figus, D. Loche, M. Atzori, V. Sarritzu, A. M. Kaczmarek, R. V. Deun, M. Saba, A. Serpe, A. Mura, M. L. Mercuri, G. Bongiovanni, P. Deplano. Light conversion control in NIR-emissive optical materials based on heterolanthanide Er
[4] S. L. Zuo, P. Chen, C. F. Pan. Mechanism of magnetic field-modulated luminescence from lanthanide ions in inorganic crystal: a review. Rare Met., 39, 1113-1126(2020).
[5] D. Tu, L. Liu, Q. Ju, Y. Liu, H. Zhu, R. Li, X. Chen. Time-resolved FRET biosensor based on amine-functionalized lanthanide-doped NaYF4 nanocrystals. Angew. Chem., 50, 6306-6310(2011).
[6] J. Liu, A. M. Kaczmarek, F. Artizzu, R. V. Deun. Ultraefficient cascade energy transfer in dye-sensitized core/shell fluoride nanoparticles. ACS Photon., 6, 659-666(2019).
[7] J. B. Zhao, L. L. Wu. Yb3+- and Er3+-doped Y2O3 microcrystals for upconversion photoluminescence and energy transfer with enhancements of near-ultraviolet emission. Rare Met., 40, 123-127(2021).
[8] J.-C. G. Bünzli, A.-S. Chauvin. Lanthanides in Solar Energy Conversion(2014).
[9] T. Kitagawa, K. Hattori, K. Shuto, M. Yasu, M. Kobayashi, M. Horiguchi. Amplification in erbium-doped silica-based planar lightwave circuits. Electron. Lett., 28, 1818-1819(1992).
[10] Y. C. Yan, A. J. Faber, H. De Waal, A. P. Polman, G. Kik. Erbium-doped phosphate glass waveguide on silicon with 4.1 dB/cm gain at 1.535 μm. Appl. Phys. Lett., 71, 2922-2924(1997).
[11] W. You, Y. Huang, Y. Chen, Y. Lin, Z. Luo. The Yb3+ to Er3+ energy transfer in YAl3(BO3)4 crystal. Opt. Commun., 281, 4936-4939(2008).
[12] J. Zhou, D. Jin. Triplet state brightens upconversion. Nat. Photonics, 12, 378-379(2018).
[13] X. Wang, R. R. Valiev, T. Y. Ohulchanskyy, H. Ågren, C. Yang, G. Chen. Dye-sensitized lanthanide-doped upconversion nanoparticles. Chem. Soc. Rev., 46, 4150-4167(2017).
[14] Q. Liu, X. Zou, Y. Shi, B. Shen, C. Cao, S. Cheng, W. Feng, F. Li. An efficient dye-sensitized NIR emissive lanthanide nanomaterial and its application in fluorescence-guided peritumoral lymph node dissection. Nanoscale, 10, 12573-12581(2018).
[15] D. Mara, F. Artizzu, P. F. Smet, A. M. Kaczmarek, K. V. Hecke, R. V. Deun. Vibrational quenching in near-infrared emitting lanthanide complexes: a quantitative experimental study and novel insights. Chem. Eur. J., 25, 15944-15956(2019).
[16] J. Zhang, C. M. Shade, D. A. Chengelis, S. Petoud. A strategy to protect and sensitize near-infrared luminescent Nd3+ and Yb3+: organic tropolonate ligands for the sensitization of Ln3+-doped NaYF4 nanocrystals. J. Am. Chem. Soc., 129, 14834-14835(2007).
[17] B. Xue, D. Wang, L. Tu, D. Sun, P. Jing, Y. Chang, Y. Zhang, X. Liu, J. Zuo, J. Song, J. Qu, E. J. Meijer, H. Zhang, X. Kong. Ultrastrong absorption meets ultraweak absorption: unraveling the energy-dissipative routes for dye-sensitized upconversion luminescence. J. Phys. Chem. Lett., 9, 4625-4631(2018).
[18] D. J. Garfield, N. J. Borys, S. M. Hamed, N. A. Torquato, C. A. Tajon, B. Tian, B. Shevitski, E. S. Barnard, Y. D. Suh, S. Aloni, J. B. Neaton, E. M. Chan, B. E. Cohen, P. J. Schuck. Enrichment of molecular antenna triplets amplifies upconverting nanoparticle emission. Nat. Photonics, 12, 402-407(2018).
[19] Z. Wang, A. Meijerink. Dye-sensitized downconversion. J. Phys. Chem. Lett., 9, 1522-1526(2018).
[20] L. Yuan, W. Lin, K. Zheng, L. He, W. Huang. Far-red to near infrared analyte-responsive fluorescent probes based on organic fluorophore platforms for fluorescence imaging. Chem. Soc. Rev., 42, 622-661(2013).
[21] T. Y. Lin, Z. J. Lian, C. X. Yao, X. Y. Sun, X. Y. Liu, Z. Y. Yan, S. M. Wu. CdSe quantum dots labeled Staphylococcus aureus for research studies of THP-1 derived macrophage phagocytic behavior. RSC Adv., 10, 260-270(2020).
[22] L. W. Runnels, S. F. Scarlata. Theory and application of fluorescence homotransfer to melittin oligomerization. Biophys. J., 69, 1569-1583(1995).
[23] R. G. McClelland, A. C. Pinder. Detection of Salmonella typhimurium in dairy products with flow cytometry and monoclonal antibodies. Appl. Environ. Microbiol., 60, 4255-4262(1994).
[24] P. Huang, W. Zheng, S. Zhou, D. Tu, Z. Chen, H. Zhu, R. Li, E. Ma, M. Huang, X. Chen. Lanthanide-doped LiLuF4 upconversion nanoprobes for the detection of disease biomarkers. Angew. Chem., 126, 1276-1281(2014).
[25] Z. Li, Y. Zhang, L. Huang, Y. Yang, Y. Zhao, G. El-Banna, G. Han. Nanoscale ‘fluorescent stone’: luminescent calcium fluoride nanoparticles as theranostic platforms. Theranostics, 6, 2380-2393(2016).
[26] B. Xu, D. Li, Z. Huang, C. Tang, W. Mo, Y. Ma. Alleviating luminescence concentration quenching in lanthanide doped CaF2 based nanoparticles through Na+ ion doping. Dalton Trans., 47, 7534-7540(2018).
[27] W. Yin, G. Tian, W. Ren, L. Yan, S. Jin, Z. Gu, L. Zhou, J. Li, Y. Zhao. Design of multifunctional alkali ion doped CaF2 upconversion nanoparticles for simultaneous bioimaging and therapy. Dalton Trans., 43, 3861-3870(2014).
[28] S. Wen, J. Zhou, P. J. Schuck, Y. D. Suh, T. W. Schmidt, D. Jin. Future and challenges for hybrid upconversion nanosystems. Nat. Photonics, 13, 828-838(2019).
[29] J. C. Boyer, F. Vetrone, L. A. Cuccia, J. A. Capobianco. Synthesis of colloidal upconverting NaYF4 nanocrystals doped with Er3+, Yb3+ and Tm3+, Yb3+ via thermal decomposition of lanthanide trifluoroacetate precursors. J. Am. Chem. Soc., 128, 7444-7445(2006).
[30] B. Xu, H. He, Z. Gu, S. Jin, Y. Ma, T. Zhai. Improving 800 nm triggered upconversion emission for lanthanide-doped CaF2 nanoparticles through sodium ion doping. J. Phys. Chem. C, 121, 18280-18287(2017).
[31] R. G. Parr, W. Yang. Density Functional Theory of Atoms and Molecules(1989).
[32] A. D. Becke. Density-functional thermochemistry. III. The role of exact exchange. J. Chem. Phys., 98, 5648-5652(1993).
[33] C. Lee, W. Yang, R. G. Parr. Development of the Colle–Salvetti correlation-energy formula into a functional of the electron density. Phys. Rev. B, 37, 785-789(1988).
[34] M. J. Frisch, G. W. Trucks, H. B. Schlegel, G. E. Scuseria, M. A. Robb, J. R. Cheeseman, G. Scalmani, V. Barone, G. A. Petersson, H. Nakatsuji, X. Li, M. Caricato, A. V. Marenich, J. Bloino, B. G. Janesko, R. Gomperts, B. Mennucci, H. P. Hratchian, J. V. Ortiz, A. F. Izmaylov, J. L. Sonnenberg, D. Williams-Young, F. Ding, F. Lipparini, F. Egidi, J. Goings, B. Peng, A. Petrone, T. Henderson, D. Ranasinghe, V. G. Zakrzewski, J. Gao, N. Rega, G. Zheng, W. Liang, M. Hada, M. Ehara, K. Toyota, R. Fukuda, J. Hasegawa, M. Ishida, T. Nakajima, Y. Honda, O. Kitao, H. Nakai, T. Vreven, K. Throssell, J. A. J. Montgomery, J. E. Peralta, F. Ogliaro, M. J. Bearpark, J. J. Heyd, E. N. Brothers, K. N. Kudin, V. N. Staroverov, T. A. Keith, R. Kobayashi, J. Normand, K. Raghavachari, A. P. Rendell, J. C. Burant, S. S. Iyengar, J. Tomasi, M. Cossi, J. M. Millam, M. Klene, C. Adamo, R. Cammi, J. W. Ochterski, R. L. Martin, K. Morokuma, O. Farkas, J. B. Foresman, D. J. Fox. Gaussian 16 Rev. C.01(2016).
[35] M. Cossi, N. Rega, G. Scalmani, V. Barone. Energies, structures, and electronic properties of molecules in solution with the C-PCM solvation model. J. Comp. Chem., 24, 669-681(2003).
[36] M. E. Casida, C. Jamorski, K. C. Casida, D. R. Salahub. Molecular excitation energies to high-lying bound states from time-dependent density-functional response theory: characterization and correction of the time-dependent local density approximation ionization threshold. J. Chem. Phys., 108, 4439-4449(1998).
[37] R. E. Stratmann, G. E. Scuseria, M. J. Frisch. An efficient implementation of time-dependent density-functional theory for the calculation of excitation energies of large molecules. J. Chem. Phys., 109, 8218-8224(1998).
[38] A. D. McLean, G. S. Chandler. Contracted Gaussian basis sets for molecular calculations. I. Second row atoms, Z=11–18. J. Chem. Phys., 72, 5639-5648(1980).
[39] R. Krishnan, J. S. Binkley, R. Seeger, J. A. Pople. Self-consistent molecular orbital methods. XX. A basis set for correlated wave functions. J. Chem. Phys., 72, 650-654(1980).
[40] M. A. Thompson. ArgusLab 4.0.1, Planaria Software LLC: Seattle, WA.
[41] C. Du, H. Wang, F. Yang, P. C. Hammel. Systematic variation of spin-orbit coupling with d-orbital filling: large inverse spin Hall effect in 3D transition metals. Phys. Rev. B, 90, 140407(2014).
[42] M. He, P. Huang, C. Zhang, F. Chen, C. Wang, J. Ma, R. He, D. Cui. A general strategy for the synthesis of upconversion rare earth fluoride nanocrystals via a novel OA/ionic liquid two-phase system. Chem. Commun., 47, 9510-9512(2011).
[43] M. Verziu, M. Serano, B. Jurca, V. I. Parvulescu, S. M. Coman, G. Scholz, E. Kemnitz. Catalytic features of Nb-based nanoscopic inorganic fluorides for an efficient one-pot conversion of cellulose to lactic acid. Catal. Today, 306, 102-110(2018).
[44] W. Zou, C. Visser, J. A. Maduro, M. S. Pshenichnikov, J. C. Hummelen. Broadband dye-sensitized upconversion of near-infrared light. Nat. Photonics, 6, 560-564(2012).
[45] W. Shao, C. K. Lim, Q. Li, M. T. Swihart, P. N. Prasad. Dramatic enhancement of quantum cutting in lanthanide-doped nanocrystals photosensitized with an aggregation-induced enhanced emission dye. Nano Lett., 18, 4922-4926(2018).
[46] J. J. Nogueira, M. Oppel, L. González. Enhancing intersystem crossing in phenotiazinium dyes by intercalation into DNA. Angew. Chem., 54, 4375-4378(2015).
[47] S. J. Strickler, R. A. Berg. Relationship between absorption intensity and fluorescence lifetime of molecules. J. Chem. Phys., 37, 814-822(1962).
[48] P. Geiregat, J. Maes, K. Chen, E. Drijvers, J. De Roo, J. M. Hodgkiss, Z. Hens. Using bulk-like nanocrystals to probe intrinsic optical gain characteristics of inorganic lead halide perovskites. ACS Nano, 12, 10178-10188(2018).
[49] W. R. Browne, J. G. Vos. The effect of deuteriation on the emission lifetime of inorganic compounds. Coord. Chem. Rev., 219, 761-787(2001).
[50] J. Liu, P. Geiregat, L. Pilia, R. Van Deun, F. Artizzu. Molecular size matters: ultrafast dye singlet sensitization pathways to bright nanoparticle emission. Adv. Opt. Mater., 9, 2001678(2021).
[51] T. Förster. 10th Spiers memorial lecture. Transfer mechanisms of electronic excitation. Discuss. Faraday Soc., 27, 7-17(1959).
[52] W. Wei, G. Chen, A. Baev, G. S. He, W. Shao, J. Damasco, P. N. Prasad. Alleviating luminescence concentration quenching in upconversion nanoparticles through organic dye sensitization. J. Am. Chem. Soc., 138, 15130-15133(2016).
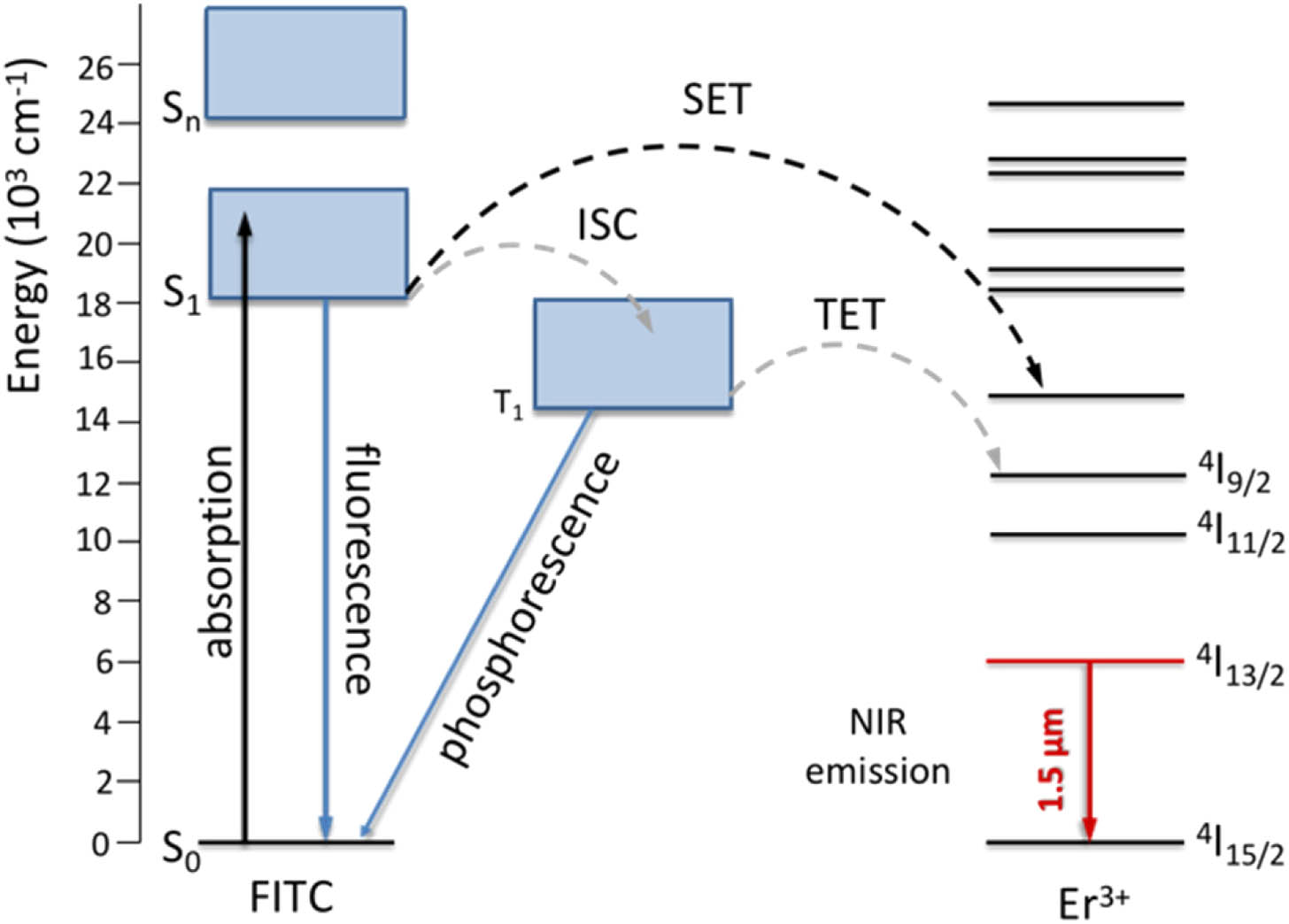
Set citation alerts for the article
Please enter your email address