
- Chinese Optics Letters
- Vol. 21, Issue 4, 041404 (2023)
Abstract
1. Introduction
Visible solid-state lasers have significantly advanced in power and beam quality, owing to the development of pump sources and crystals[1,2]. The commercially available laser diode (LD)-pumped sources, having the advantages of compactness, high power, and cost-effectiveness, are favored by researchers. Of the many approaches to generate visible lasers,
In particular, the lasers at 696 and 698 nm in the deep red region have served many different purposes. For instance, the method of obtaining ultraviolet (UV) laser by direct frequency doubling of the deep red laser is more compact and has a higher conversion efficiency than the two-stage nonlinear process to generate a UV laser, which is more suitable for obtaining higher-power UV lasers[22–26]. Additionally, the 696 nm lasers can be used for the spectral analysis of the methanol chlorophyll equation[27], the enzymatic reaction steps of the photoreduction of prochlorophyll-ester[28], and the improvement of the picture resolution of the Cr(III)tris-oxalato complex[29]. The lasers at 698 nm are important not only for research into high-precision atomic clocks[30], but also for nondestructive, real-time, or portable potato quality measurements[31].
To the best of our knowledge, the maximum output power at around 698 nm was reported to be 1.5 W using an optically pumped semiconductor laser (OPSL) as the pump source[32]. However, there are currently few reports of
Sign up for Chinese Optics Letters TOC. Get the latest issue of Chinese Optics Letters delivered right to you!Sign up now
In this paper, we demonstrate a compact and efficient InGaN LD-pumped
2. Experiment Setup
The schematic setup of the LD-pumped
Figure 1.Schematic experimental setup of the LD-pumped Pr3+:YLF CW laser.
Figure 2 displays the transmission curves of the IM and OC. The IM has high transmission from 400 to 630 nm and high reflection (
Figure 2.Transmission curve of IM and OC.
We inserted a 2-mm thickness Lyot filter with a Brewster angle into the laser resonator to select the wavelength. The operation performance of the single-wavelength 696.6 and 698.6 nm laser was optimized, respectively. To measure the output power, filters with high red transmittance and high blue reflectance were placed behind the output to filter out the pump light. The laser spectra in the experiment were measured by an optical spectral analyzer (Ocean-Optics HR4000+), with a resolution of about 0.25 nm.
3. Result and Discussion
The
Figure 3.Partial energy level scheme of Pr3+:YLF crystal.
Figure 4 depicts the laser output characteristics. The spectra were measured at the maximum output power with emission peaks at 698.6 and 696.6 nm, respectively [see Figs. 4(b)–4(d)]. According to the previous studies on spectral analysis,[4] the emission cross sections at 696 and 698 nm are smaller relative to 721 nm (
Figure 4.Laser output performance at 698.6 and 696.6 nm. (a), (c) Experimental input–output relationship and simulation results; (b), (d) laser emission spectra of 698.6 and 696.6 nm.
Figure 5.(a), (b) Measured corresponding M2 factors of 696.6 and 698.6 nm, respectively; (c), (d) power stabilities of lasers at 696.6 and 698.6 nm.
To better understand the experimental results, we performed some theoretical simulations on the relationship between output power and absorbed pump power. For a four-level laser system, the input–output characteristics can be determined by[36]
For a circular Gaussian laser beam, by introducing the parameter
λ (nm) | σe (10-20 cm2) | Isat (mW/µm2) | ϖp (µm) | γ | T (%) |
---|---|---|---|---|---|
696 | 1.51 | 5.106 | 75 | 0.0155 | 0.42 |
698 | 9.79 | 0.785 | 108 | 0.023 | 1.55 |
Table 1. Parameters Used in the Simulation of Lasers at 698 and 696 nm
As can be seen from Table 1, the laser emission cross section at 698 nm is
4. Conclusion
In summary, we reported LD-pumped
References
[1] C. Kränkel, D. T. Marzahl, F. Moglia, G. Huber, P. W. Metz. Out of the blue: semiconductor laser pumped visible rare-earth doped lasers. Laser Photonics Rev., 10, 548(2016).
[2] H. Tanaka, S. Kalusniak, M. Badtke, M. Demesh, N. V. Kuleshov, F. Kannari, C. Kränkel. Visible solid-state lasers based on Pr3+ and Tb3+. Prog. Quantum. Electron., 84, 100411(2022).
[3] A. Richter, E. Heumann, E. Osiac, G. Huber, W. Seelert, A. Diening. Diode pumping of a continuous-wave Pr3+-doped LiYF4 laser. Opt. Lett., 29, 2638(2004).
[4] T. Gün, P. Metz, G. Huber. Power scaling of laser diode pumped Pr3+:LiYF4 cw lasers: efficient laser operation at 522.6 nm, 545.9 nm, 607.2 nm, and 639.5 nm. Opt. Lett., 36, 1002(2011).
[5] Y. Y. Ren, Z. M. Cui, L. F. Sun, C. Wang, H. L. Liu, Y. J. Cai. Laser emission from low-loss cladding waveguides in Pr:YLF by femtosecond laser helical inscription. Chin. Opt. Lett., 20, 122201(2022).
[6] F. Cornacchia, A. Di Lieto, M. Tonelli, A. Richter, E. Heumann, G. Huber. Efficient visible laser emission of GaN laser diode pumped Pr-doped fluoride scheelite crystals. Opt. Express, 16, 15932(2008).
[7] D. Pabœuf, O. Mhibik, F. Bretenaker, P. Goldner, D. Parisi, M. Tonelli. Diode-pumped Pr:BaY2F8 continuous-wave orange laser. Opt. Lett., 36, 280(2011).
[8] P. Camy, J. L. Doualan, R. Moncorgé, J. Bengoechea, U. Weichmann. Diode-pumped Pr3+:KY3F10 red laser. Opt. Lett., 32, 1462(2007).
[9] A. S. Rao, T. Miike, K. Miyamoto, T. Omatsu. Optical vortex lattice mode generation from a diode-pumped Pr3+:LiYF4 laser. J. Opt., 23, 075502(2021).
[10] A. Sottile, Z. Zhang, S. Veronesi, D. Parisi, A. D. Lieto, M. Tonelli. Visible laser operation in a Pr3+:LiLuF4 monocrystalline fiber grown by the micro-pulling-down method. Opt. Mater. Express, 6, 1964(2016).
[11] M. Fibrich, H. Jelínková. Power-scaled Pr:YAlO3 laser at 747 and 720 nm wavelengths. Laser Phys. Lett., 10, 035801(2013).
[12] M. Fechner, F. Reichert, N.-O. Hansen, K. Petermann, G. Huber. Crystal growth, spectroscopy, and diode pumped laser performance of Pr,Mg:SrAl12O19. Appl. Phys. B, 102, 731(2011).
[13] M. Fibrich, J. Šulc, H. Jelínková. Pr:YAlO3 laser performance enhancement by operating the laser close to liquid helium temperatures. Opt. Laser Technol., 148, 107653(2022).
[14] H. Chen, H. Uehara, H. Kawase, R. Yasuhara. Efficient Pr:YAlO3 lasers at 622 nm, 662 nm, and 747 nm pumped by semiconductor laser at 488 nm. Opt. Express, 28, 3017(2020).
[15] L. B. Zhou, J. Y. Zou, W. X. Zheng, T. Zhang, B. Xu, X. D. Xu, A. A. Lyapin, J. Xu. More than 2.3 W diode-pumped quasi-continuous-wave Pr, Mg:SrAl12O19 bulk laser and the first demonstration of Co:ZnSe-based passively Q-switched deep red laser at 724 nm. Opt. Laser Technol., 145, 107471(2022).
[16] B. Xu, Z. Liu, H. Xu, Z. P. Cai, C. Zeng, S. Huang, Y. Yan, F. Wang, P. Camy, J. L. Doualan, A. Braud, R. Moncorge. Highly efficient InGaN-LD-pumped bulk Pr:YLF orange laser at 607 nm. Opt. Commun., 305, 332(2013).
[17] Y. J. Cheng, B. Xu, B. Qu, S. Y. Luo, H. Yang, H. Y. Xu, Z. P. Cai. Comparative study on diode-pumped continuous wave laser at 607 nm using differently doped Pr3+:LiYF4 crystals and wavelength tuning to 604 nm. Appl. Opt., 53, 7898(2017).
[18] B. Xu, P. Camy, J. L. Doualan, Z. P. Cai, R. Moncorge. Visible laser operation of Pr3+-doped fluoride crystals pumped by a 469 nm blue laser. Opt. Express, 19, 1191(2011).
[19] B. Qu, Q. Huang. Watt-level diode-pumped continuous-wave Pr:LiYF4 laser at 670 nm and simultaneous dual-wavelength operation at 639 and 670 nm. Appl. Opt., 59, 3033(2020).
[20] Z. Liu, Z. P. Cai, B. Xu, S. L. Huang, C. H. Zeng, Y. Yan, F. J. Wang, H. Y. Xu, J. L. Doualan, P. Camy, R. Moncorgé. Continuous-wave laser emission of Pr:LiYF4 at 695.8 nm. IEEE Photon. Technol. Lett., 26, 675(2014).
[21] Z. Liu, Z. P. Cai, S. L. Huang, C. H. Zeng, Z. Y. Meng, Y. K. Bu, Z. Q. Luo, B. Xu, H. Y. Xu, C. C. Ye, F. Stareki, P. Camy, R. Moncorgé. Diode-pumped Pr3+:LiYF4 continuous-wave deep red laser at 698 nm. J. Opt. Soc. Am. B, 30, 302(2013).
[22] V. Ostroumov, W. Seelert, L. Hunziker, C. Ihli, A. Richter, E. Heumann, G. Huber. UV generation by intracavity frequency doubling of an OPS-pumped Pr:YLF laser with 500 mW of cw power at 360 nm. Proc. SPIE, 6451, 645103(2007).
[23] N. Niu, S. Pu, Q. Chen, Q. Chen, Y. Wang, Y. Zhao, W. Wu, Q. Zheng. 302 nm continuous wave generation by intracavity frequency doubling of a diode-pumped Pr:YLF laser. Appl. Opt., 57, 9798(2018).
[24] Z. Liu, Z. P. Cai, B. Xu, C. H. Zeng, S. L. Huang, F. J. Wang, Y. Yan, H. Y. Xu. Continuous-wave ultraviolet generation at 349 nm by intracavity frequency doubling of a diode-pumped Pr:LiYF4 laser. IEEE Photon. J., 5, 1500905(2013).
[25] A. Srinivasa Rao, N. Apurv Chaitanya, G. K. Samanta. High-power, high repetition-rate, ultrafast fibre laser based source of DUV radiation at 266 nm. OSA Contin., 2, 99(2019).
[26] Y. S. Zhang, J. Y. Zou, W. X. Zheng, K. Feng, B. Xu, Z. F. Yu. Watt-level continuous-wave intracavity frequency-doubled Pr:YLF-LBO laser at 320 nm. Chin. Opt. Lett., 19, 091406(2021).
[27] R. J. Ritchie. Consistent sets of spectrophotometric chlorophyll equations for acetone, methanol and ethanol solvents. Photosyn. Res., 89, 27(2006).
[28] D. J. Heyes, C. N. Hunter. Making light work of enzyme catalysis: protochlorophyllide oxidoreductase. Trends Biochem. Sci., 30, 642(2005).
[29] G. L. J. A. Rikken, E. Raupach. Enantioselective magnetochiral photochemistry. Nature, 405, 932(2000).
[30] M. G. Tarallo, N. Poli, M. Schioppo, D. Sutyrin, G. M. Tino. A high-stability semiconductor laser system for a 88 Sr-based optical lattice clock. Appl. Phys. B, 103, 17(2011).
[31] Z. Zhou, S. W. Zeng, X. Y. Li, J. Zheng. Nondestructive detection of blackheart in potato by visible/near infrared transmittance spectroscopy. J. Spectrosc., 2015, 786709(2015).
[32] P. W. Metz, F. Reichert, F. Moglia, S. Müller, D. T. Marzahl, C. Kränkel, G. Huber. High-power red, orange, and green Pr3+:LiYF4 lasers. Opt. Lett., 39, 3193(2014).
[33] B. Xu, Y. J. Cheng, B. Qu, S. Y. Luo, H. Y. Xu, Z. P. Cai, P. Camy, J. L. Doualan, R. Moncorgé. InGaN-LD-pumped Pr3+:LiYF4 continuous-wave deep red lasers at 697.6 and 695.8 nm. Adv. Opt. Photonics, 67, 146(2015).
[34] S. Y. Luo, B. Xu, S. W. Cui, H. Chen, Z. P. Cai, H. Y. Xu. Diode-pumped continuous-wave dual-wavelength c-cut Pr3+:LiYF4 laser at 696 and 719 nm. Appl. Opt., 54, 10051(2015).
[35] L. Esterowitz, F. J. Bartoli, R. E. Allen, D. E. Wortman, C. A. Morrison, R. P. Leavitt. Energy levels and line intensities of Pr3+ in LiYF4. Phys. Rev. B, 19, 6442(1979).
[36] P. Laporta, M. Brussard. Design criteria for mode size optimization in diode-pumped solid-state lasers. IEEE J. Quantum Electron., 27, 2319(1991).
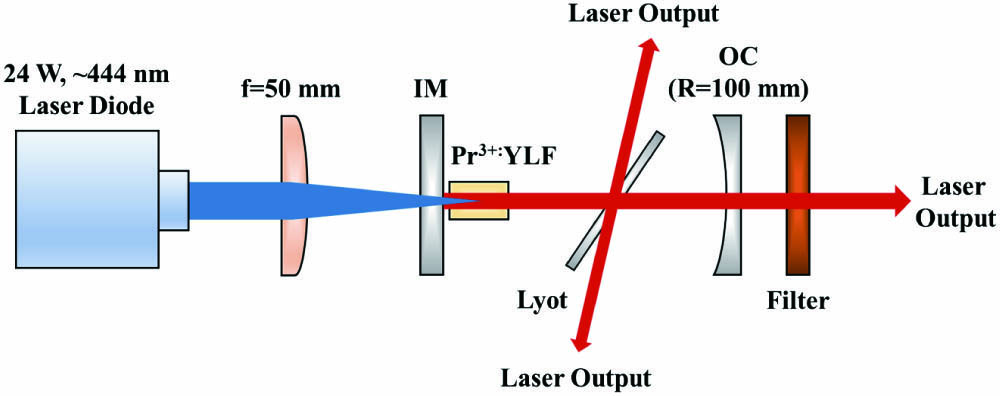
Set citation alerts for the article
Please enter your email address