Abstract
We report the successful synthesis and characterization of a novel 1111-type magnetic semiconductor (Ba1?xNax)F(Zn1?xMnx)Sb (0.05 ≤x ≤ 0.175) with tetragonal ZrSiCuAs-type structure, which is isostructural to the layered iron-based superconductor La(O,F)FeAs. Na substitutions for Ba and Mn substitutions for Zn introduce carriers and local magnetic moments, respectively. Ferromagnetic interaction is formed when Na and Mn are codoped, demonstrating that local magnetic moments are mediated by carriers. Iso-thermal magnetization shows that the coercive field is as large as ~ 12 000 Oe, which is also reflected in the large split between the temperature-dependent magnetization in zero-field-cooling and field-cooling condition. AC susceptibility under zero field demonstrates that samples evolve into spin-glass state below spin freezing temperatureTf. The measurements of temperature-dependent resistivity indicate that (Ba1?xNax)F(Zn1?xMnx)Sb exhibits semiconducting behaviour.We report the successful synthesis and characterization of a novel 1111-type magnetic semiconductor (Ba1?xNax)F(Zn1?xMnx)Sb (0.05 ≤x ≤ 0.175) with tetragonal ZrSiCuAs-type structure, which is isostructural to the layered iron-based superconductor La(O,F)FeAs. Na substitutions for Ba and Mn substitutions for Zn introduce carriers and local magnetic moments, respectively. Ferromagnetic interaction is formed when Na and Mn are codoped, demonstrating that local magnetic moments are mediated by carriers. Iso-thermal magnetization shows that the coercive field is as large as ~ 12 000 Oe, which is also reflected in the large split between the temperature-dependent magnetization in zero-field-cooling and field-cooling condition. AC susceptibility under zero field demonstrates that samples evolve into spin-glass state below spin freezing temperatureTf. The measurements of temperature-dependent resistivity indicate that (Ba1?xNax)F(Zn1?xMnx)Sb exhibits semiconducting behaviour.Introduction
Due to the potential applications in spintronics devices, diluted magnetic semiconductors (DMSs) that simultaneously combine the spin and charge degrees of electrons have received extensive attentions[1-6]. In 1990s, the III–V DMS (Ga,Mn)As was successfully prepared by low temperature molecular beam epitaxy (LT-MBE) method. The Curie temperature
has reached as high as ~200 K[7-9], which is much higher than those of some II–VI DMSs, such as (Zn,Mn)Te[10] and (Cd,Mn)Te[11]. To explain the magnetic mechanism of III–V DMSs, Dietlet al. proposed a mean-field Zener's model that described the ferromagnetic interactions between the local magnetic moments and carriers, and predicted that
of some DMSs may reach room temperature if more Mn atoms are doped[12,13]. Subsequently, Reedet al. reported that
of (Ga,Mn)N was up to ~370 K[14]. The application of DMSs in spintronic devices will be practical once the Curie temperature of some DMSs are above room temperature. However, the development of III–V DMSs has also encountered some limitations. For example, in (Ga,Mn)As, magnetic atoms Mn easily enter the interstitial positions of the lattice, which makes it difficult to accurately determine the actual amount of doped Mn atoms. Meanwhile, the substitutions of Mn for Ga introduce magnetic moments and carriers simultaneously, which constrains us to study their individual contribution to the formation of the ferromagnetic ordering. In addition, films cannot be used for microscopic probes that are based on bulk materials, such as nuclear magnetic resonance (NMR), neutron scattering and muon spin relaxation (μSR)[5,15-17]. On that account, the study of bulk DMSs with decoupled charge and spin doping are worthy of attentions.
Recently, many bulk Zn-based DMSs derived from Fe-based superconductors have been reported. Foremost, according to the pioneer theory work of Maseket al.[18], Denget al.[16] prepared a new 111-type DMS Li(Zn,Mn)As, which is isostructural to Fe-based superconductor LiFeAs[19]. This is the first bulk DMS that carriers and magnetic moments could be controlled independently. In I–II–V semiconductor LiZnAs, doping excessive Li introduces carriers and Mn substitutions for Zn introduce local moments, and the Curie temperature
is ~50 K. Until now, the highest Curie temperature recorded is a 122-type DMS (Ba,K)(Zn,Mn)2As2 with
up to ~230 K[20,21], which has surpassed that of (Ga,Mn)As. In addition, Dinget al.[17] synthesized a 1111-type DMS (La,Ba)(Zn,Mn)AsO with
up to ~40 K.μSR measurements have proved that the ferromagnetic ordering of these DMSs is intrinsic rather than caused by magnetic clusters or impurities. Moreover,μSR measurements reveal that Li(Zn,Mn)As, (Ba,K)(Zn,Mn)2As2 and (La,Ba)(Zn,Mn)AsO share the same mechanism of magnetic origin as that of (Ga,Mn)As[16,17,20,22]. That is to say, searching for more new DMSs materials will help us to understand the general mechanism of magnetic ordering in all DMSs, and direct us to optimize the choices of materials.
In this paper, we report the successful fabrication of a new 1111-type magnetic semiconductor, (Ba1−xNax)F(Zn1−xMnx)Sb (x = 0.05, 0.075, 0.1, 0.125, 0.15, and 0.175), via doping Na into Ba sites and Mn into Zn sites in the parent compound BaFZnSb to introduce hole carriers and local magnetic moments, respectively. BaFZnSb shares the same crystal structure with that of LaOZnAs and LaOCuS, which have been reported to be the parent semiconductors of 1111-type DMSs (La,Ba)O(Zn,Mn)As (
~ 40 K)[17] and (La,Sr)O(Cu,Mn)S (
~ 200 K)[23], respectively. Comparing with oxides, the ionic radius of fluoride is smaller and its electronegativity is stronger, which results in constituting stronger ionic bonds[24]. The Weiss temperatureθ of (Ba1−xNax)F(Zn1−xMnx)Sb is up to ~ 16 K forx = 0.175, which is a sign of ferromagnetic interaction, and followed by a magnetic glassy transition below
~ 14 K.
Experiments
We synthesized the polycrystalline specimens of (Ba1−xNax)F(Zn1−xMnx)Sb through the solid-state reaction method. High-purity starting materials Ba (99.2%, Alfa Aesar), BaF2 (99.99%, Aladdin), Na (99.7%, Aladdin), Zn (99.9%, Alfa Aesar), Mn (99.95%, Alfa Aesar) and Sb (99.999%, Prmat) were mixed according to stoichiometric proportions and were placed in alumina crucibles before sealing in evacuated silica tubes. The mixture was heated slowly to 200 °C and held for 10 h, then heated to 750 °C and held for another 10 h, followed by furnace cooling to room temperature. After that, the intermediate products were grounded, pressed into pellets, placed in alumina crucibles, sealed in evacuated silica tubes again and then reheated to 750 °C for 30 h. To protect the contamination from air and H2O, all operations except the sealing of silica tubes were executed in a glove box filled with high-purity Ar (O2 and H2O < 0.1 ppm).
The crystal structures of the samples were characterized at room temperature by a powder X-ray diffractometer (Model EMPYREAN) using monochromatic Cu-Kα1 radiation withλ(Kα1) = 1.540598 Å. The detailed information of lattice constants and unit cell volume was calculated by Rietveld refinement method using the GSAS-II software package[25]. The DC magnetization measurements were performed on a quantum design magnetic property measurement system (MPMS). The AC susceptibility measurements were measured on a quantum design physical property measurement system (PPMS). The electrical resistivity measurements were conducted on sintered pellets by the four-probe method.
Results and discussion
InFig. 1(a), we show the powder X-ray diffraction patterns of polycrystals (Ba1−xNax)F(Zn1−xMnx)Sb withx = 0.00, 0.05, 0.075, 0.1, 0.125, 0.15, and 0.175, respectively. The Bragg peaks can be well indexed by a ZrSiCuAs-type tetragonal structure with space group P4/nmm[26], which is isostructural to the 1111-type superconductor La(F,O)FeAs[27], indicating that Na substitution for Ba and Mn substitution for Zn have no influence on the tetragonal crystal structure. InFig. 1(b), we show the Rietveld refinement result of (Ba0.875Na0.125)F(Zn0.875Mn0.125)Sb. The resultant weighted reliable factor
is 7.962%, indicating the samples are in good quality. With doping levelx increasing, some small peaks of ZnSb impurities marked as * inFig. 1(a) were observed. ZnSb impurities are non-magnetic, thus they will not affect the discussion of magnetism in the following.
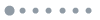
Figure 1.(Color online) (a) The polycrystal powder X-ray diffraction patterns of (Ba1−xNax)F(Zn1−xMnx)Sb (x = 0.00, 0.05, 0.075, 0.10, 0.125, 0.15, and 0.175). Traces of impurities ZnSb are marked as stars (*). (b) The Rietveld refinement result of (Ba0.875Na0.125)F(Zn0.875Mn0.125)Sb. Inset shows the tetragonal ZrCuSiAs-type crystal structure of parent compound BaFZnSb. (c) Lattice parametersa andc versus doping levelx of (Ba1−xNax)F(Zn1−xMnx)Sb (x = 0.00, 0.05, 0.075, 0.10, 0.125, 0.15, and 0.175). (d) The unit cell volume of (Ba1−xNax)F(Zn1−xMnx)Sb (x = 0.00, 0.05, 0.075, 0.10, 0.125, 0.15, and 0.175).
As shown inFig. 1(b), the parent compound BaFZnSb has a layered crystal structure, which consists of two layers in the ab plane: one is [BaF]+ layers (BaF4 tetrahedra) and the other is [ZnSb]– layers (ZnSb4 tetrahedra)[28]. These layers are conventionally regarded as the tetragonal fluorite and anti-fluorite structure types separately[28], and they are stacked alternately along thec-axis. The lattice parameters obtained from Rietveld refinement are shown inFig. 1(c). The lattice parameters of parent compound BaFZnSb area = 4.43605 Å andc = 9.81786 Å, which is close to previous reported values ofa = 4.4384 Å andc = 9.7789 Å[28]. Meanwhile, with doping levelx increasing,a monotonically increases from 4.43720 to 4.44271 Å, andc continuously decreases from 9.80767 to 9.78259 Å, repectively. This should be ascribed to the fact that the ionic radius of Na+ (1.02 Å) is smaller than that of Ba2+ (1.35 Å), but the ionic radius of Mn2+ (0.83 Å) is larger than that of Zn2+ (0.74 Å). The monotonical behaviours of lattice parametersa andc with doping levelx demonstrate the successful doping of Na into Ba sites and Mn into the Zn sites, respectively. InFig. 1(d), we find that the unit cell volume doesn't vary monotonically with increasing doping levelx. This is due to the fact that lattice parametersa andc behave in opposite direction.
Table Infomation Is Not Enable
We show DC magnetization (M) measurements of the parent phase BaFZnSb and (Ba0.95Na0.05)FZnSb under field-cooling condition with an external field
= 100 Oe inFig. 2(a). We find that the magnetization for both samples is very small, ~10–8 emu/mg, confirming the characteristic of paramagnetism. By using the Curie-Weiss law to fit the data, we get the values of Weiss temperatures as
and
K, respectively. This also demonstrates that both BaFZnSb and (Ba0.95Na0.05)FZnSb are in paramagnetic ground state. That is to say, doping only carriers can not introduce any type of magnetic phase transition. This is similar to the situation in SrF(Zn,Cu)Sb[29], where doping only Cu into SrFZnSb does not induce any magnetic ordering.
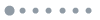
Figure 2.(Color online) (a) The temperature dependence of DC magnetization for parent phase BaFZnSb and (Ba0.95Na0.05)FZnSb under field-cooling mode in an external magnetic field of 100 Oe. The data (open circles) are the data dots, and the solid lines are the Curie-Weiss fitting results. (b) The temperature dependent magnetization (M) for (Ba1−xNax)F(Zn1−xMnx)Sb (x = 0.05, 0.075, 0.10, 0.125, 0.15 and 0.175) in both zero-field-cooling (ZFC) and field-cooling (FC) procedures under an external magnetic field of 100 Oe. Inset shows the enlarged M(T) curves for all specimens at low temperature. Arrow marks
forx = 0.10. (c) The plot of
versusT for (Ba1−xNax)F(Zn1−xMnx)Sb (x = 0.05, 0.075, 0.10, 0.125, 0.15 and 0.175) under FC condition. Arrows mark the Weiss temperatures. Inset shows the enlarged plot of
versusT for all of specimens below 30 K. (d) Iso-thermal magnetization for (Ba1−xNax)F(Zn1−xMnx)Sb (x = 0.05, 0.075, 0.10, 0.125, 0.15 and 0.175) at 2 K. Inset shows the enlargedM(H) curves for all of specimens under an external magnetic field
from –20 000 to 20 000 Oe.
InFig. 2(b), we show the temperature-dependent magnetization (M) in both zero-field-cooling (ZFC) and field-cooling (FC) procedures under an applied external field
= 100 Oe for (Ba1−xNax)F(Zn1−xMnx)Sb (x = 0.05, 0.075, 0.1, 0.125, 0.15 and 0.175). There is an obvious increase for the magnetic moment at low temperature. Also, ZFC and FC curves abruptly split at a temperature defined as
(as marked by the arrow in the inset ofFig. 2(b)) for all doping levels, which is related to the pinning of the domain wall[30]. InFig. 2(b), for (Ba0.9Na0.1)F(Zn0.9Mn0.1)Sb, the split between FC and ZFC curves is at
~ 16 K, and the maximum of ZFC curve is at
~ 13 K. On the other hand, the magnetic moments (
) at base temperature of 2 K under FC mode increase from 0.0038
/Mn forx = 0.05 to 0.0112
/Mn forx = 0.10, and then decrease to 0.0092
/Mn forx = 0.15. In the inset ofFig. 2(b), we can clearly observe the variation of magnetic moments under low temperature for specimens withx = 0.05, 0.075, 0.1, 0.125, 0.15 and 0.175, respectively. The increasing
forx ≤ 0.1 may be attributed to the formation and enhancement of ferromagnetic interaction.
decreases forx ≥ 0.125, which might be generated by the competition between local ferromagnetic coupling through the indirect exchange interaction and antiferromagnetic coupling caused by direct exchange interaction existing in Mn atoms at nearest-neighbour (N.N.) Zn sites. This is also a piece of evidence that too many Mn atoms doping is detrimental to the ferromagnetic interaction, as shown in many types of DMSs, such as (La,Sr)(Zn,Mn)SbO[31] and (Ca,Na)(Zn,Mn)2Sb2[32]. The probability that two Mn atoms are at N.N. sites satisfies
. For example, for the doping level ofx = 0.1, for a Mn atom finding one Mn atom at its N.N. sites is
, andP increases to 36.85% forx = 0.15. That is, as the Mn doping levelx continues to rise, the enhanced antiferromagnetic interaction suppresses the ferromagnetic interation.
When the temperature is much higher than the Curie temperature, the magnetic susceptibility generally satisfies the Curie-Weiss law,
, where
is a temperature-independent component,C is the Curie constant andθ is Weiss temperature. InFig. 2(c), we plot
versus temperature. The intersections of the fitting lines and thex-axis are the Weiss temperaturesθ. All values ofθ are positive, further identifying the establishment of ferromagnetic interaction between Mn atoms. Moreover, Zn/Mn-Sb bond length is longer than that of Zn/Mn-As, and Sb-Zn/Mn-Sb bond angleα is further away from the idealα value in non-distorted ideal tetrahedron as that of As-Zn/Mn-As[33]. On that account, compared with that of (Ba,K)F(Zn,Mn)As[34], the Weiss temperature of our samples is lower. In addition, we also apply the formula
to obtain effective magnetic moment
, and put these numbers inTable 1.
InFig. 2(d), we present the iso-thermal magnetization at 2 K for (Ba1−xNax)F(Zn1−xMnx)Sb (x = 0.05, 0.075, 0.1, 0.125, 0.15 and 0.175). Clear hysteresis loops are observed, which indicates the presence of ferromagnetic interaction. With the increasing of doping levelx, the coercive field
decreases. In particular, the largest
is up to ~12 000 Oe, which is also reflected in the large differences between the measurements of temperature-dependent magnetization (M) in ZFC and FC condition at
. The large coercive field
is similar to that in (La,Ba)(Zn,Mn)AsO[17] and (Ba,K)F(Zn,Mn)As[34], but much larger than that in Li(Zn,Mn)As[16] and Cu2(Zn,Mn)(Sn,Al)Se4[35]. Comparing the crystal structures of two kinds of materials mentioned above, we find that the coercive fields seem to be much larger in crystals with layered structure. We list all parameters obtained above inTable 1.
We observe that the magnetization has not even reached saturation at 2 K under the maximum external magnetic field as high as 5 T inFig. 2(d), which is a characteristic of spin-glass systems. Therefore, we perform AC susceptibility with varying frequenciesf under zero field for (Ba0.9Na0.1)F(Zn0.9Mn0.1)Sb. The real part
and imaginary part
of the AC susceptibility are shown inFigs. 3(a) and3(b), respectively. As the frequency increases, the cusp temperature,
, shifts to higher temperature on both real part
and imaginary part
, and the overall magnitude of real part
slightly decreases. We apply the equation
to describe thef-dependence of
[36]. The value ofK obtained inFig. 3(c) is ~ 0.077, which is within the range between 0.005 to 0.08 of conventional spin-glass systems[37], indicating our samples evolve into spin-glass state below
. Similar behaviour has also been observed in other DMSs, such as Ba(Zn,Cu,Mn)2As2[38] and (Ba,K)(Zn,Mn)2Sb2[39]. The frustrated magnetic moments of spin-glass behaviour may be ascribed to the competition between ferromagnetic and antiferromagnetic interactions among magnetic Mn atoms[40].
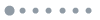
Figure 3.(Color online) The (a) real part
and (b) imaginary part
of AC susceptibility with varying frequenciesf under zero field for (Ba0.9Na0.1)F(Zn0.9Mn0.1)Sb. (c) A frequency dependence of spin freezing temperature
for (Ba0.9Na0.1)F(Zn0.9Mn0.1)Sb.
Electrical transport measurement is another important characterization method of magnetic semiconductors. InFig. 4, we perform temperature-dependent resistivityρ for (Ba1−xNax)F(Zn1−yMny)Sb (x = 0.00,y = 0.00;x = 0.05,y = 0.00;x = 0.05,y = 0.05;x = 0.075,y = 0.075;x = 0.10,y = 0.10;x = 0.125,y = 0.125;x = 0.15,y = 0.15;x = 0.175,y = 0.175). Both of the parent compound BaFZnSb and (Ba0.95Na0.05)FZnSb where only carriers are introduced show metallic behaviour. The semiconducting behaviour is displayed for all samples with substitutions of (Ba,Na) and (Zn,Mn) simultaneously. Remarkably, the resistivity increases monotonically with the doping levelx increasing (except forx = 0.175), similar to that in (La,Ca)(Zn,Mn)SbO[41]. The main cause for that is that carriers are scattered by magnetic fluctuations caused by doped Mn atoms. Similar phenomenon has also been reported in (Ga,Mn)As[42].
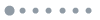
Figure 4.(Color online) Temperature-dependent resistivity measurements for (Ba1−xNax)F(Zn1−yMny)Sb (x = 0.00,y = 0.00;x = 0.05,y = 0.00;x = 0.05,y = 0.05;x = 0.075,y = 0.075;x = 0.10,y = 0.10;x = 0.125,y = 0.125;x = 0.15,y = 0.15;x = 0.175,y = 0.175).
Summary
To summarize, we successfully synthesized a new 1111-type fluoride-antimonide magnetic semiconductor (Ba1−xNax)F(Zn1−xMnx)Sb (x = 0.05, 0.075, 0.10, 0.125, 0.15 and 0.175) via Na substitutions for Ba supplying carriers and Mn substitutions for Zn introducing local magnetic moments, respectively. Forx = 0.175, the Weiss temperature is up to ~ 16 K. The iso-thermal magnetization measurements at 2 K for (Ba1−xNax)F(Zn1−xMnx)Sb show clear hysteresis loops, and the coercive field
is as large as 12 000 Oe, similar to that in (La,Ba)(Zn,Mn)AsO[17] and (Ba,K)F(Zn,Mn)As[34]. Furthermore, the AC susceptibility measurements show that the system evolves into spin-glass state below
. The temperature-dependent resistivity measurements demonstrate that all specimens with Na and Mn codoped display semiconducting behaviour. The Zn-based magnetic semiconductor (Ba1−xNax)F(Zn1−xMnx)Sb, Fe-based surperconductor LaFeAsO and antiferromagnet LaMnAsO have the same ZrSiCuAs-type tetragonal structure, which is possible to make multifunctional heterojunctions. The magnetic semiconductors in fluoride compounds have shown some difference from oxide compounds, and provide new ideas for the development of new materials.
Acknowledgements
The work was supported by the Key R&D Program of Zhejiang Province, China (2021C01002) and NSF of China (No. 12074333).
References
[1] H Ohno. Making nonmagnetic semiconductors ferromagnetic. Science, 281, 951(1998).
[2] T Dietl, H Ohno. Dilute ferromagnetic semiconductors: Physics and spintronic structures. Rev Mod Phys, 86, 187(2014).
[3] T Dietl. A ten-year perspective on dilute magnetic semiconductors and oxides. Nat Mater, 9, 965(2010).
[4] T Dietl, A Bonanni, H Ohno. Families of magnetic semiconductors — an overview. J Semiconduct, 40, 080301(2019).
[5] Y L Gu, S L Guo, F L Ning. Progress on microscopic properties of diluted magnetic semiconductors by NMR andμSR. J Semicond, 40, 081506(2019).
[6] G Q Zhao, Z Deng, C Q Jin. Advances in new generation diluted magnetic semiconductors with independent spin and charge doping. J Semicond, 40, 081505(2019).
[7] H Munekata, H Ohno, S von Molnar et al. Diluted magnetic III-V semiconductors. Phys Rev Lett, 63, 1849(1989).
[8] M Wang, R P Campion, A W Rushforth et al. Achieving high curie temperature in (Ga, Mn)As. Appl Phys Lett, 93, 132103(2008).
[9] L Chen, X Yang, F H Yang et al. Enhancing the Curie temperature of ferromagnetic semiconductor (Ga, Mn)As to 200 K via nanostructure engineering. Nano Lett, 11, 2584(2011).
[10] D Ferrand, J Cibert, C Bourgognon et al. Carrier-induced ferromagnetic interactions in p-doped Zn1−xMnxTe epilayers. J Cryst Growth, 214/215, 387(2000).
[11] A Haury, A Wasiela, A Arnoult et al. Observation of a ferromagnetic transition induced by two-dimensional hole gas in modulation-doped CdMnTe quantum wells. Phys Rev Lett, 79, 511(1997).
[12] T Dietl, H Ohno, F Matsukura et al. Zener model description of ferromagnetism in zinc-blende magnetic semiconductors. Science, 287, 1019(2000).
[13] T Dietl, H Ohno, F Matsukura. Hole-mediated ferromagnetism in tetrahedrally coordinated semiconductors. Phys Rev B, 63, 195205(2001).
[14] M L Reed, N A El-Masry, H H Stadelmaier et al. Room temperature ferromagnetic properties of (Ga, Mn)N. Appl Phys Lett, 79, 3473(2001).
[15] S L Guo, F L Ning. Progress of novel diluted ferromagnetic semiconductors with decoupled spin and charge doping: Counterparts of Fe-based superconductors. Chin Phys B, 27, 097502(2018).
[16] Z Deng, C Q Jin, Q Q Liu et al. Li(Zn,Mn)As as a new generation ferromagnet based on a 1-2-5 semiconductor. Nat Commun, 2, 1(2011).
[17] C Ding, H Y Man, C Qin et al. (La1-xBax)(Zn1-xMnx)AsO: A two dimensional “1111” diluted magnetic semiconductor in bulk form. Phys Rev B, 88, 041102(2013).
[18] J Masek, J Kudrnovský, F MȢca et al. Dilute moment n-type ferromagnetic semiconductor Li(Zn, Mn)As. Phys Rev Lett, 98, 067202(2007).
[19] X C Wang, Q Q Liu, Y X Lv et al. The superconductivity at 18 K in LiFeAs system. Solid State Commun, 148, 538(2008).
[20] K Zhao, Z Deng, X C Wang et al. New diluted ferromagnetic semiconductor with Curie temperature up to 180 K and isostructural to the n122o iron-based superconductors. Nat Commun, 4, 1442(2013).
[21] K Zhao, B J Chen, G Q Zhao et al. Ferromagnetism at 230 K in (Ba0.7K0.3)(Zn0.85Mn0.15)2As2 diluted magnetic semiconductor. Chin Sci Bull, 59, 2524(2014).
[22] S R Dunsiger, J P Carlo, T Goko et al. Spatially homogeneous ferromagnetism of (Ga, Mn)As. Nat Mater, 9, 299(2010).
[23] X J Yang, Y K Li, C Y Shen et al. Sr and Mn co-doped LaCuSO: A wide band gap oxide diluted magnetic semiconductor withTC around 200 K. Appl Phys Lett, 103, 022410(2013).
[24] Agulyansky A. Main principals of the chemistry of tantalum and niobium fluoride compounds. Elsevier, 2004
[25] B H Toby, R B Von Dreele. GSAS-II: The genesis of a modern open-source all purpose crystallography software package. J Appl Crystallogr, 46, 544(2013).
[26] V Johnson, W Jeitschko. ZrCuSiAs: A “filled” PbFCl type. J Solid State Chem, 11, 161(1974).
[27] Y Kamihara, T Watanabe, M Hirano et al. Iron-based layered superconductor La[O1–xFx] FeAs (x = 0.05–0.12) withTc = 26 K. J Am Chem Soc, 130, 3296(2008).
[28] H Kabbour, L Cario, F Boucher. Rational design of new inorganic compounds with the ZrSiCuAs structure type using 2D building blocks. J Mater Chem, 15, 3525(2005).
[29] L C Fu, Y L Gu, S L Guo et al. Ferromagnetism in fluoride-antimonide SrF(Zn1–2xMnxCux)Sb with a quasi two dimensional structure. J Magn Magn Mater, 483, 95(2019).
[30] J Dho, W S Kim, N H Hur. Reentrant spin glass behavior in Cr-doped perovskite manganite. Phys Rev Lett, 89, 027202(2002).
[31] H J Zhang, R F Zhang, L C Fu et al. (La1−xSrx)(Zn1−xMnx)SbO: A novel 1111-type diluted magnetic semiconductor. Acta Physica Sinica, 70, 107501(2021).
[32] Y L Gu, H J Zhang, R F Zhang et al. A novel diluted magnetic semiconductor (Ca, Na)(Zn, Mn)2Sb2 with decoupled charge and spin doping. Chin Phys B, 29, 057507(2020).
[33] L C Fu, Y L Gu, G X Zhi et al. Drastic improvement of Curie temperature by chemical pressure in N-type diluted magnetic semiconductor Ba(Zn, Co)2As2. Sci Rep, 11, 7652(2021).
[34] B J Chen, Z Deng, W M Li et al. New fluoride-arsenide diluted magnetic semiconductor (Ba, K)F(Zn, Mn)As with independent spin and charge doping. Sci Rep, 6, 36578(2016).
[35] G X Zhi, S L Guo, R F Zhang et al. Cu2(Zn, Mn)(Sn, Al)Se4: A diluted magnetic semiconductor with decoupled charge and spin doping. J Magn Magn Mater, 536, 168064(2021).
[36] X F Liu, S Matsuishi, S Fujitsu et al. Spin-glass-like behavior of CaNi1−xMnxGe. Phys Rev B, 84, 214439(2011).
[37] P N Lekshmi, G R Raji, M Vasundhara. Re-entrant spin glass behaviour and magneto-dielectric effect in insulating Sm2NiMnO6 double perovskite. J Mater Chem C, 1, 6565(2013).
[38] H Y Man, S L Guo, Y Sui et al. Ba(Zn1−2xMnxCux)2As2: A bulk form diluted ferromagnetic semiconductor with Mn and Cu codoping at Zn sites. Sci Rep, 5, 15507(2015).
[39] S Yu, G Q Zhao, Y Peng et al. (Ba, K)(Zn, Mn)2Sb2: A new type of diluted magnetic semiconductor. Crystals, 10, 690(2020).
[40] K Binder, A P Young. Spin glasses: Experimental facts, theoretical concepts, and open questions. Rev Mod Phys, 58, 801(1986).
[41] W Han, K Zhao, X C Wang et al. Diluted ferromagnetic semiconductor (LaCa)(ZnMn)SbO isostructural to “1111” type iron pnictide superconductors. Sci China Phys Mech Astron, 56, 2026(2013).
[42] T Jungwirth, J Sinova, J Mašek et al. Theory of ferromagnetic (III, Mn)V semiconductors. Rev Mod Phys, 78, 809(2006).