Fig. 1. Diarylethene units used in single-molecule switches.(
a) Diarylethene photoisomerization mechanism. (
b) Potential energy curves of the molecular switching. The switching process is initiated by an excitation to the first excited state. (
c) Diarylethene bridged between the electrode ends. (
d) Molecular structures with special design for single-molecule switches. (
e) A graphene-diarylethene-graphene single-molecule switch. (
f) Self-assembled monolayer devices with diarylethene units. (
g) Molecular isomerization under external controls of electrochemical potential and light irradiation. Figure reproduced with permission from: (a) ref.
24, American Chemical Society; (b) ref.
26, American Physical Society; (c) ref.
27, American Chemical Society; (d) ref.
28, John Wiley and Sones; (e) ref.
7, American Association for the Advancement of Science; (f) ref.
30, American Chemical Society; (g) ref.
32, under a Creative Commons Attribution 3.0 Unported Licence.
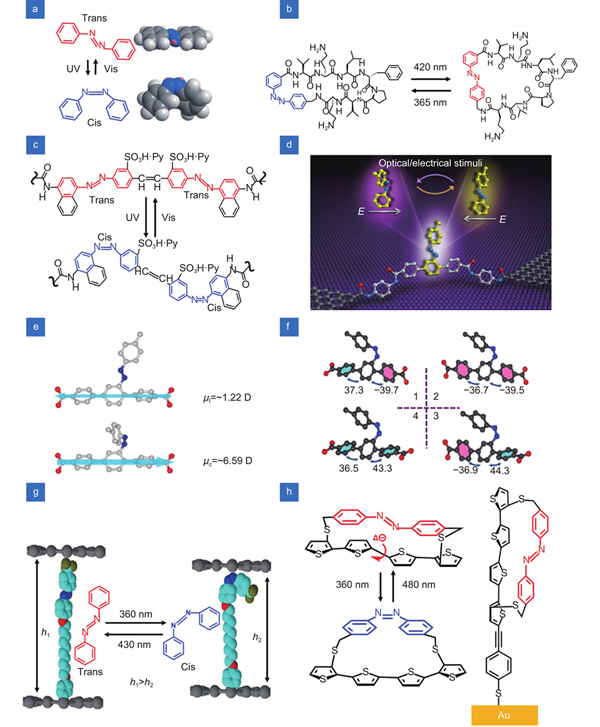
Fig. 2. Azobenzene units used in single-molecule switches. (
a) Structures of
trans and
cis isomers of azobenzene. (
b) Azobenzene as the core unit in the macromolecule. (
c) Chemical structures of molecules whose configurational change of the azobenzene unit occurs directly in the molecular backbone. (
d) Schematic of azobenzene as the side group of bridging molecule in the junction. (
e) Dipole projection on the molecular backbone. The arrow denotes the direction of the dipole projection. (
f) Four different conformational states due to the asymmetry caused by the introduction of additional azobenzene side group in
cis form in the terphenyl backbone. (
g) Vertical distance between two graphene electrodes is regulated by the conformational changes in aryl azobenzene molecules with light irradiation. (
h) Vertical tunneling self-assembled monolayer device with an azobenzene derivative on Au electrode. Figure reproduced with permission from: (a, c) ref.
33, (b) ref.
34, (f) ref.
36, John Wiley and Sons; (d, e) ref.
35, under a Creative Commons Attribution 4.0 International License; (g) ref.
37, Springer Nature; (h) ref.
38, American Chemical Society.
Fig. 3. Other units used in single-molecule switches.(
a) DHP/CPD single-molecule junction. (
b) DHA/VHF single-molecule junction. (
c) SP/MC single-molecule junction. (
d) Schematic diagram of a vertical tunneling molecular switch with rGO thin films as the transparent top contact and the molecular structures of DHA and VHF. (
e) Single-molecule switches with chirality molecules. The spin-polarization direction of the current switches with the chirality inversion. (
f) Schematic diagram of SP SAMs in EGaIn/Ga
2O
3//SAM/Au
TS junctions in their open and closed forms. Figure reproduced with permission from: (a) ref.
39, American Chemical Society; (b) ref.
40, under a Creative Commons Attribution 4.0 International License; (c) ref.
41, American Chemical Society; (d) ref.
43, John Wiley and Sons; (e) refs.
19, 44, under a Creative Commons Attribution 4.0 International License; (f) ref.
42, American Chemical Society.
Fig. 4. Photoconductance of single-molecule devices. (
a) Schematic illustration of the Au/NH-PTCDI-NH/Au junction under light irradiation. (
b) SAM-templated addressable nanogap devices comprised of AminoPyr or SC18. (
c) Schematic presentation of the charge transport of a molecular junction affected by the excitation. (
d) Bonding geometry of a porphyrin-C
60 dyad molecule in the gold-ITO tunnel junction. (
e) Schematic diagram of carbon/bilayer/carbon tunneling junctions. (
f) Energy level diagram for a BTB–AQ bilayer junction. Figure reproduced with permission from: (a, c) ref.
47, American Chemical Society; (b) ref.
50, under a Creative Commons Attribution 4.0 International License; (d) ref.
49, American Chemical Society; (e, f) ref.
51, John Wiley and Sons.
Fig. 5. Photo-assisted transport mechanism in tunnelling junctions. (
a) A vacuum gold tunnelling junction. (
b) Plasmonic enhancement of the electromagnetic field in the junctions. (
c) Schematic representation of the mechanically controllable break fiber junction chip. The inset shows a zoomed view of the suspended fiber/Cr/Au bridge. (
d) Strong shift of the electrode energy level in imidazole single-molecule junctions caused by photon absorption. Figure reproduced with permission from: (a) ref.
54, Springer Nature; (b) ref.
55, American Chemical Society; (c, d) ref.
56, The Royal Society of Chemistry.
Fig. 6. “Hot electron” effect of single-molecule devices. (
a) Schematic demonstration of an illuminated metal-molecule-metal junction with 4,4′-bipyridine (BP) molecule. (
b) Nonradiative decay of SPP with generated hot electrons and holes. (
c) Nonequilibrium distribution of hot electrons and holes in biased junctions. (
d) Experimental setup and strategy to map hot-carrier energy distributions. (
e) Schematic illustration of the real-time observation of the plasmon-induced chemical reaction. Figure reproduced with permission from: (a) ref.
59, American Chemical Society; (b–d) ref.
62, (e) ref.
63, American Association for the Advancement of Science.
Fig. 7. Photovoltaic effect in single-molecule devices. (
a) A GaAs-molecule-Au molecular junction. (
b) Schematic band diagram for the illuminated metal-molecule-semiconductor junction under reverse biases. (
c)
I–
V characteristics of the Au-GaAs junction. Figure reproduced with permission from: (a, b) ref.
66, American Chemical Society; (c) ref.
67, The Royal Society of Chemistry.
Fig. 8. Electroluminescence of plasmon. (
a) Photon emission induced by inelastic tunneling through a nano-gap between a sharp Au tip and an Au substrate. (
b) Schematic diagram and mechanism diagram of how hot electrons excite plasmon electroluminescence. (
c) Illustration of the molecular tunneling junction with a SAM of SC
n. (
d) Blinking of plasmon sources obtained from molecular tunneling junction with a SC
12 SAM. (
e) Corresponding spectra of plasmon electroluminescence excited at different biases. Figure reproduced with permission from: (a) ref.
71, American Chemical Society; (b) ref.
72, (c–e) ref.
73, Springer Nature.
Fig. 9. Electroluminescence from molecules in a STM nanocavity. (
a) A single ZnPc molecule in a STM nanocavity, where the Au (111) substrate is covered with sodium chloride. (
b) Schematic demonstration of the luminescence mechanism for a neutral ZnPc. (
c) STM-induced light emission spectrum (black line) of the ZnPc linear tetramer. (
d) Phosphorescence scanning tunnel luminescence map of the PTCDA/NaCl/Ag (111) system. (
e) Schematic images of the exciton formation mechanism; the arrows represent electrons. Arrows up and down represent the direction of electron spin. (
f) Peak characteristics of fluorescence and phosphorescence. Figure reproduced with permission from: (a, b) ref.
77, American Association for the Advancement of Science; (c) ref.
80, American Physical Society; (d−f) ref.
82, Springer Nature.
Fig. 10. Coupling of molecular electroluminescence and plasmon nanocavity. (
a) Two different junction structures: on top of the molecule or in close proximity to the molecule. (
b) STM-induced luminescence of the situation that the tip is in close proximity to the molecule which cause Fano resonance. (
c) Excitation of molecular fluorescence through intermolecular energy transfer. (
d) Schematic illustration of the process of intermolecular energy transfer. (
e) Exciton splitting diagram for different coherent dipole–dipole coupling modes. Figure reproduced with permission from: (a, b) ref.
83, under a Creative Commons Attribution 4.0 International License; (c, d) ref.
86, (e) ref.
87, Springer Nature.
Fig. 11. Electroluminescence in single-molecule junctions. (
a) A polythiophene molecular wire suspended between the metal substrate and the tip of STM. (
b) Fluorescent junctions with different emitting units suspending between the substrate and the tip of the STM by oligothiophene chains. (
c) A single graphene nanoribbon junction. (
d) Device structure of the nanotube–molecule–nanotube junction. (
e) Chemical structure of the molecule, which consists of a central 2,6-dibenzylamino core-substituted NDI chromophore (blue), two OPE rods (red) and phenanthrene anchor units (green). (
f) Energy-level model with the HOMO and LUMO molecular orbitals of the OPE and NDI subunits. Figure reproduced with permission from: (a) ref.
90, American Physical Society; (b) ref.
91, American Chemical Society; (c) ref.
92, American Chemical Society; (d–f) ref.
93, Springer Nature.