
- Chinese Optics Letters
- Vol. 19, Issue 12, 123701 (2021)
Abstract
Keywords
1. Introduction
Terahertz (THz) waves have high penetration through non-metallic and non-polar materials, and they have low photon energy, so are safer for biological targets and human operators. Because of these special properties, both the two-dimensional (2D) and three-dimensional (3D) THz imaging techniques have gained widespread attention in biomedical fields[
A number of methods for 3D imaging with THz radiation have also been proposed and demonstrated. One of main modalities is the THz computed tomography (CT)[
Diffraction tomography (DT) is one of the common tomography methods that accounts for the diffraction effect[
Sign up for Chinese Optics Letters TOC. Get the latest issue of Chinese Optics Letters delivered right to you!Sign up now
In this Letter, we proposed a CW-THz DT system by using a coherent single-frequency THz laser and the array detector to directly record the off-axis digital hologram. The setup is simple and easy to operate. By rotating the object, the 2D scattered fields of the object at each rotation angle can be obtained by the digital holographic reconstruction method. With regard to the 3D reconstruction algorithm of the THz DT, to the best of our knowledge, the filtered backpropagation (FBPP) method is first introduced to produce the volume data in the space domain and to achieve high-quality 3D RI measurement. Compared with the regular FDI algorithm, there is no need to do complex interpolation in the Fourier domain, and the experimental results demonstrate the validity of the method with high fidelity.
2. Principle of the Filtered Backpropagation Method
On the assumption of the scalar wave diffraction theorem, consider a monochromatic plane wave
In order to solve Eq. (1), we can make use of Green’s function and apply the Rytov approximation, so the scattered field can be obtained as[
Then, a direct relation between the Fourier transform (FT) of the scattered field and the FT of the scattering potential of the object can be deduced, which is commonly called the FDT[
To reconstruct exact
In Eq. (5), there are two exponential terms inside the integral formula. The first one is a transfer function, which is depth-dependent due to the parameter
It is noted that the FBPP algorithm generally requires scattered data measured from view angles in [
Practically, in order to achieve the complex diffracted fields (including the amplitude and phase distributions) of the object for one illumination angle
3. Experimental Setup and Results
An experimental CW THz DT system based on off-axis Fresnel digital holography was built as depicted in Fig. 1 to collect the scattered field of the sample with different rotation angles. The THz source was an optically pumped far-IR gas laser (295-FIRL; Edinburgh Instruments Ltd., UK) with a central wavelength of 118.83 µm (2.52 THz) and a maximum power of 500 mW. The emitted THz beam was expanded and collimated by two off-axis parabolic mirrors (PM1,
Figure 1.Schematic of the experimental configuration of continuous-wave terahertz diffraction tomography (CW THz DT). PM1 and PM2, off-axis parabolic mirrors; BS, THz beam splitter; M, gold-coated mirror; RS, rotational stage.
To validate the proposed method, we first used a single polystyrene (PS) foam sphere with a diameter of 7.44 mm. The RI of the PS foam was
Figure 2.Reconstructed results of digital holography for single polystyrene (PS) foam sphere: (a1), (b1) holograms with and without the object at 0°; (c1), (d1) reconstructed amplitude and wrapped phase images of (a1); (a2)–(d2) reconstructed phase images at 0°, 45°, 90°, and 180°; (e1) phase profiles of the black dotted line in (a2)–(d2); (e2) result of (e1) after alignment.
The reconstructed complex amplitudes by digital holography with various angles were then processed to obtain the scattered field. The scattering potential distributions of the sample were reconstructed by using Eq. (5), where
Figures 3(a1)–3(c1) are the reconstructed results by the FDI method, showing the obtained RI distributions at cross sections
Figure 3.Reconstructed refractive index (RI) profiles of DT for a single foam sphere: (a1)–(c1), (a2)–(c2) 3D RI profiles at cross sections x–y, y–z, and x–z by FDI and FBPP algorithm, respectively; (d) RI profiles of the red dotted line in (c1) and (c2), and ideal values obtained by the THz-TDS system.
Figure 4.Amplitude of the spectrum distribution of (a) FDI and (b) FBPP on the logarithmic scale along the fx–fy, fx–fz, and fy–fz cross sections, respectively.
To demonstrate further the applicability of THz DT on non-axisymmetric samples, two glued PS foam spheres were placed horizontally on the rotary stage, and then the sample was rotated around the gravity center of one sphere to obtain the scattered field with various rotation angles. The reconstructed RI distributions of the foam spheres at cross sections
Figure 5.RI distributions of DT for two foam spheres: (a)–(c) 3D RI profiles at cross sections x–y, y–z, and x–z; (d) volume rendering of 3D RI profiles (see Visualization 1).
4. Summary and Discussion
In summary, we have realized CW THz DT combined with digital holography, and the digital holograms are recorded directly by the array detector at various angles through rotating the samples. The configuration is simple and easy to operate. With regard to the 3D reconstruction algorithm, the FBPP algorithm is adopted to achieve 3D RI distributions of the PS foam spheres. The average RI value has only 0.16% difference from the RI value measured by the THz-TDS system. The reconstructed results have high fidelity compared with results obtained by the FDI algorithm. It verifies the feasibility of the proposed method.
Note that in the reconstructed RI tomograms in Figs. 3 and 5, some values are quite different from their surroundings along the Y axis, which is the result of the “missing apple core” problem. It can be improved by the iterative algorithm with non-negative constraints. Furthermore, to promote THz DT further, RI matching or the multiple scattering non-linear model must be applied to reconstruct high-RI samples. Our view is that THz DT can be an effective method for non-destructive testing and quantitative measurement of the complex RI of complex samples in the future, which can be combined with other THz wide-field phase-contrast imaging methods.
References
[1] D. A. Lima, J. Song, X. R. Li, A. Portieri, Y. C. Shen, J. A. Zeitler, H. Lin. Review of terahertz pulsed imaging for pharmaceutical film coating analysis. Sensors, 20, 1441(2020).
[2] L. Valzania, Y. Zhao, L. Rong, D. Wang, M. Georges. THz coherent lensless imaging. Appl. Opt., 58, G256(2019).
[3] D. M. Mittleman. Twenty years of terahertz imaging. Opt. Express, 26, 9417(2018).
[4] X. Xu, Y. Wu, T. He, Y. Li, F. Hu, H. Liang, C. Yang, H. Zhong. Metamaterials-based terahertz sensor for quick diagnosis of early lung cancer. Chin. Opt. Lett., 15, 111703(2017).
[5] J. P. Guillet, B. Recur, L. Frederique, B. Bousquet, L. Canioni, I. Manek-Hönninger, P. Desbarats, P. Mounaix. Review of terahertz tomograph techniques. J. Infrared Milli. THz Waves, 35, 382(2014).
[6] J. B. Perraud, A. Chopard, J. P. Guillet, P. Gellie, A. Vuillot, P. Mounaix. A versatile illumination system for real-time terahertz imaging. Sensors, 20, 3993(2020).
[7] F. Xing, D. Liu, L. Xing, S. Shen, Z. Yang, J. Li, K. Wang. Generation of a meter-scale THz diffraction-free beam based on multiple cascaded lens-axicon doublets: detailed analysis and experimental demonstration. Opt. Express, 28, 36873(2020).
[8] J. B. Perraud, J. Bou Sleiman, B. Recur, H. Balacey, F. Simoens, J. P. Guillet, P. Mounaix. Liquid index matching for 2D and 3D terahertz imaging. Appl. Opt., 55, 9185(2016).
[9] L. Chen, Y. Wang, D. Xu, Y. Ren, Y. He, C. Li, C. Zhang, L. Tang, C. Yan, J. Yao. Terahertz computed tomography of high-refractive-index objects based on refractive index matching. IEEE Photon. J., 10, 5900813(2018).
[10] A. Brahm, A. Wilms, M. Tymoshchuk, C. Grossmann, G. Notni, A. Tünnermann. Optical effects at projection measurements for terahertz tomography. Opt. Las. Technol., 62, 49(2014).
[11] M. Born, E. WolfPrinciple of Optics(1999).
[12] W. Gorski, W. Osten. Tomographic imaging of photonic crystal fibers. Opt. Lett., 32, 1977(2007).
[13] C. Liu, M. Malek, I. Poon, L. Jiang, A. Siddiquee, C. Sheppard, A. Roberts, H. Quiney, D. G. Zhang, X. Yuan, J. Lin, C. Depeursinge, P. Marquet, S. S. Kou. Simultaneous dual-contrast three-dimensional imaging in live cells via optical diffraction tomography and fluorescence. Photon. Res., 7, 1042(2019).
[14] J. Oh, J. S. Ryu, M. Lee, J. Jung, S. Han, H. J. Chung, Y. Park. Three-dimensional label-free observation of individual bacteria upon antibiotic treatment using optical diffraction tomography. Biomed. Opt. Express, 11, 1257(2020).
[15] K. Kim, J. Yoon, Y. Park. Large-scale optical diffraction tomography for inspection of optical plastic lenses. Opt. Lett., 41, 934(2016).
[16] V. Balasubramani, A. Kus, H. Y. Tu, C. J. Cheng, M. Baczewska, W. Krauze, M. Kujawińska. Holographic tomography: techniques and biomedical application. Appl. Opt., 60, B65(2021).
[17] S. Wang, B. Ferguson, D. Abbott, X. Zhang. T-ray imaging and tomography. J. Bio. Phys., 29, 247(2003).
[18] S. Wang, X. Zhang. Pulsed terahertz tomograhy. J. Phys. D, 37, R1(2004).
[19] S. H. Ding, Q. Li, Y. D. Li, Wang Qi. Cotinuous-wave terahertz digital holography by use of a pyroelectric array camera. Opt. Lett., 36, 1993(2010).
[20] M. S. Heimbeck, H. O. Everitt. Terahertz digital holographic imaging. Adv. Opt. Photon., 12, 1(2020).
[21] L. Valzania, T. Feurer, P. Zolliker, E. Hack. Terahertz ptychograpy. Opt. Lett., 43, 543(2018).
[22] A. J. Devaney. Inverse-scattering theory within the Rytov approximation. Opt. Lett., 6, 374(1981).
[23] P. Müller, M. Schürmann, J. Guck. The theory of diffraction tomography(2015).
[24] A. J. Devaney. A filtered backpropagation algorithm for diffraction tomography. Ultrason. Imaging, 4, 336(1982).
[25] J. Kostencka, T. Kozacki, A. Kuś, B. Kemper, M. Kujawińska. Holographic tomography with scanning of illumination: space-domain reconstruction for spatially invariant accuracy. Biomed. Opt. Express, 7, 4086(2016).
[26] K. Kim, J. Yoon, S. Shin, S. Lee, S. Yang, Y. Park. Optical diffraction tomography techniques for the study of cell pathophysiology. J. Biomed. Photon. Eng., 2, 020201(2016).
[27] Z. Zhao, H. Zhang, C. Ma, C. Fan, H. Zhao. Comparative study of phase unwrapping algorithms based on solving the Poisson equation. Meas. Sci. Technol., 31, 065004(2020).
[28] P. Zhang, D. Liu, A. Yang, J. Zhu. Optimization of compensation for high spatial frequency in distorted wavefront using optical phase conjugation. Chin. Opt. Lett., 17, 070901(2019).
[29] B. Zhong, Y. Zhang, J. Hu, Z. Jin, Z. Wang, L. Sun. Improved autofocus method for human red blood cell images. Appl. Opt., 58, 8031(2019).
[30] J. Lim, A. Goy, M. H. Shoreh, M. Unser, D. Psaltis. Learning tomography assessed using Mie theory. Phys. Rev. Appl., 9, 034027(2018).
[31] J. Cheng, J. Luo. Tikhonov-regularization-based projecting sparsity pursuit method for fluorescence molecular tomography reconstruction. Chin. Opt. Lett., 18, 011701(2020).
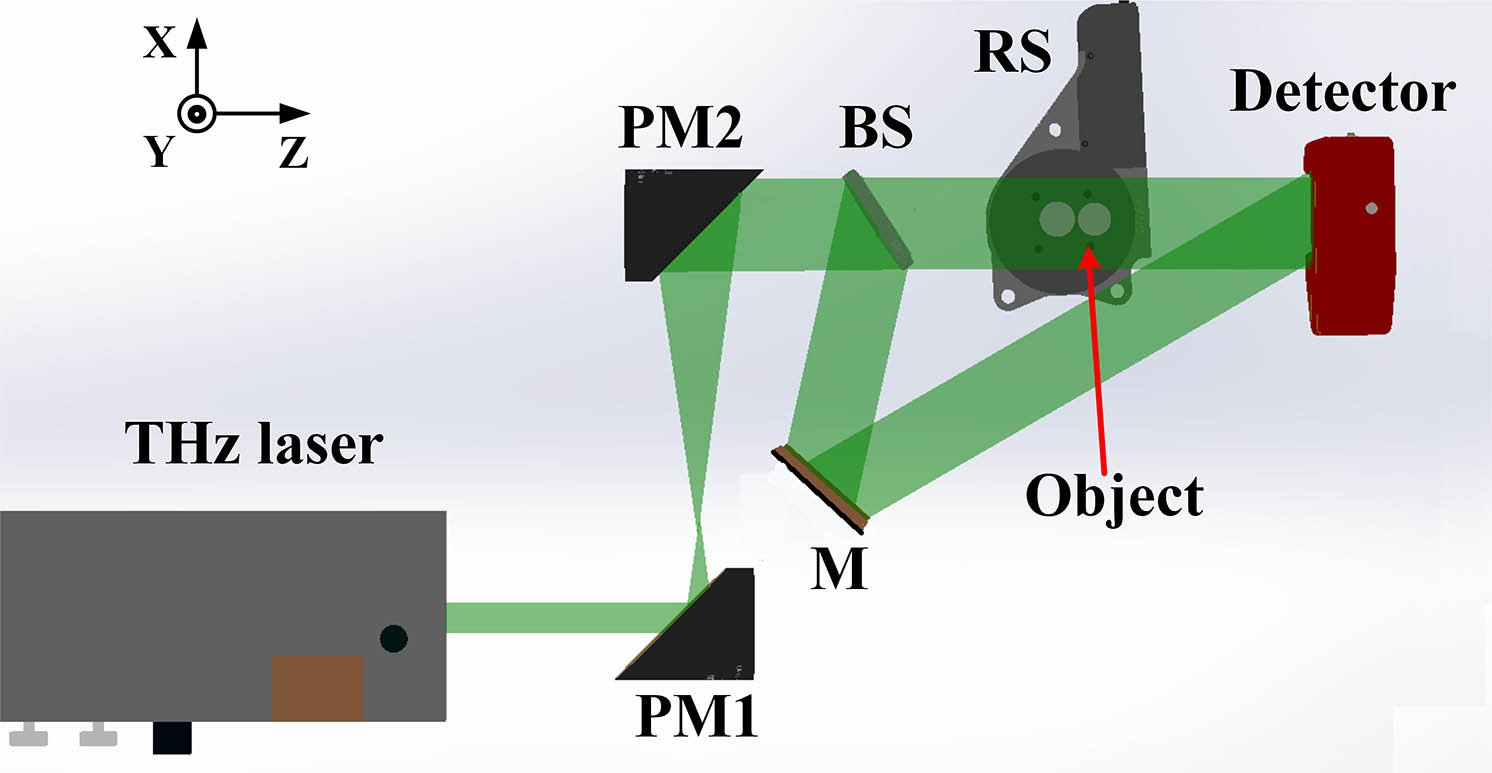
Set citation alerts for the article
Please enter your email address