
- Chinese Optics Letters
- Vol. 20, Issue 1, 011401 (2022)
Abstract
1. Introduction
Ultrafast lasers play key roles in scientific fields such as optical clocks, optical precision measurement, and materials processing[
Apart from the widely researched pulsating solitons, the intermittency is rarely researched in ultrafast lasers, manifesting as the state where the optical wave keeps the metastable state for a long time while transiently entering the striking state, followed by the recovery of the metastable state[
In this Letter, the coherent DS intermittency, whose evolution strongly relies on the gain dynamics, is observed experimentally in the fiber laser. For lower pump power, the DS switches between the weak and extreme pulsations without loss of coherence. By increasing the pump power, the laser outputs the bi-stable DS, i.e., the DS that switches between two coherent states with different wavelengths and intensities. The laser is prone to annihilate into the random mode-locking state with high pump power. Experimental results demonstrate the dominance of slow-gain dynamics on the intermittency. Further mechanisms of the intermittency remain to be explored by the accurate numerical simulation that contains the slow dynamics of the gain medium.
Sign up for Chinese Optics Letters TOC. Get the latest issue of Chinese Optics Letters delivered right to you!Sign up now
2. Experimental Setup
The schematic of the fiber laser is shown in Fig. 1. The cavity comprises an 8 m Er-doped fiber (EDF) and a 4 m single-mode fiber (SMF) with net dispersions of 21.5 and
Figure 1.Schematic of the fiber laser. EDF, Er-doped fiber; ISO, isolator; OC, output coupler; CNT, carbon nanotube; PC, polarization controller; LD, laser diode; WDM, wavelength division multiplexer; DCF, dispersion compensation fiber; OSC, oscilloscope; RFA, radio frequency analyzer; OSA, optical spectrum analyzer; AC, autocorrelator.
3. Experimental Results
Tuning the pump power slightly above the threshold of mode locking, the intermittent state is obtained as shown in Fig. 2. The burrs on the time-averaged spectrum [measured by an optical spectrum analyzer (OSA)] indicate special transient events during the DS evolution [see Fig. 2(a)]. The average width of the DS is 7.13 ps according to the autocorrelation trace in Fig. 2(b), wherein the smooth profile validates the coherence of the mode-locked pulse that is distinct from the noise-like pulse. The non-stationary mode-locking state is confirmed via modulating the sidebands on the radio frequency (RF) spectrum in Fig. 2(c). The normalized peak intensity of the time-stretch signals versus roundtrips (RTs) is shown in Fig. 2(d). Different from the conventional pulsating DS with stable oscillating amplitude and period[
Figure 2.Mode-locking state at the pump power of 25.35 mW. (a) Spectrum measured by OSA, (b) autocorrelation trace, (c) RF spectrum, and (d) peak intensity evolution of the normalized DFT signals over 85,000 RTs.
To reveal the details of intermittency, real-time spectral evolution is plotted in Fig. 3. As shown in Figs. 3(a) and 3(b), the weak pulsation transforms into a transition state with slight fluctuations, followed by the extreme pulsation with strong spectral peaks induced by the self-phase-modulation (SPM) [Figs. 3(a) and 3(b)]. The periods of the weak and extreme pulsations are 327 and 338 RTs, corresponding to frequencies of 52.7 and 51 kHz, respectively, in agreement with the measured value of 49 kHz from the RF spectrum [Fig. 2(c)]. Despite the exotic evolution of the DS, the average spectrum over 16,000 RTs in Fig. 3(c) agrees qualitatively with that measured by OSA. The SPM-induced transient spectral broadening during the extreme pulsation [see Fig. 3(d)] accounts for the burrs of the spectrum measured by the OSA [see Fig. 2(a)]. One can note that two basic stages, i.e., weak and extreme pulsations, emerge alternatively, which is distinct from the pulsating DS. What is more, the coherent state is maintained during the switching between basic stages. In this regard, the unique phenomenon is named the coherent DS intermittency.
Figure 3.Shot-to-shot evolution of the pulse at the pump power of 25.35 mW. (a) Intensity evolution of normalized DFT signals over 16,000 RTs, (b) spatial-spectral evolution, (c) averaged spectrum measured by DFT, (d) local enlargement of the marked area, and (e) single-shot spectra at three RTs.
The dependence of the intermittency on the pump power is displayed in Fig. 4. By fixing the PC while gradually increasing the pump power, the intermittent pulsation gradually disappears [see Figs. 4(a)–4(c)]. The DS reaches the quasi-bi-stable state at the pump power of 28.2 mW, switching between the high and low levels without pulsation [Figs. 4(d)–4(f)]. The duration of the low-level state for bi-stable DS decreases from 5241 to 2524 RTs as the pump power increases from 28.20 to 31.95 mW. Further increasing the pump power to 32.85 mW, the bi-stable DS annihilates into a stochastic state of the random mode-locking.
Figure 4.Spectral intensity evolution of the normalized DFT signals over 80,000 RTs at different pump powers: (a) 24.39 mW, (b) 25.35 mW, (c) 26.32 mW, (d) 28.20 mW, (e) 29.21 mW, and (f) 31.95 mW.
Detailed properties of the bi-stable DS at the pump power of 31.95 mW, including average and real-time properties, are illustrated in Fig. 5. The OSA measured spectrum has steep edges with burrs induced by intermittency [Fig. 5(a)]. The sidebands on the RF spectrum indicate the 1.14 kHz modulating frequency of the intermittency [Fig. 5(b)]. To reveal the wavelength shifting of the spectrum, the weighted central wavelength (WCW) of each single-shot spectrum is calculated by
Figure 5.Pulse state at the pump power of 31.95 mW. (a) Optical spectrum measured by OSA, (b) RF spectrum, (c) center wavelength and intensity evolution, and (d) shot-to-shot evolution.
In a previous work[
The random mode-locking state at the pump power of 32.85 mW is displayed in Fig. 6. Comparing the results with the those of intermittency, we summarize three similarities between them: (i) there are two peaks in the spectrum corresponding to the random mode locking at wavelengths of 1558.2 and 1558.99 nm [Fig. 6(a)], whose separation of 0.79 nm is similar to the wavelength jumping step of the bi-stable DS (
Figure 6.Random mode locking at the pump power of 32.85 mW. (a) Optical spectrum measured by OSA, (b) shot-to-shot evolution of the time-stretch signals, and (c) enlargement of the marked areas in (b).
A clear fact is that the slow-gain dynamics, i.e., the recovery and depletion of the population inversion of the
4. Discussion
In our work, despite the distinct evolutionary behaviors at different pump powers, the coherent DS intermittency is always composed of two basic stages, e.g., either weak and extreme pulsations at lower pump power or two quasi-stationary DSs with different wavelengths and intensities at higher pump power. It is not easy to understand why the basic stage of the intermittency transforms from the pulsating DS to the quasi-stationary DS with increasing pump power, however, the results of the pulsating DS in Ref. [12] give us enlightening thoughts. Peng et al. found that the pulsating DS emerges at lower pump power, while the stationary DS favors higher pump power, and such an experimental phenomenon is reproduced by numerical simulations with the average-field equation, wherein the pulsating DS is found to be related to the quintic terms of the gain. In this regard, we think the distinct basic stages of the intermittency at different pump powers have a similar origination to that in Ref. [12].
Spontaneous switching between basic stages is an important difference between DS intermittency and conventional pulsating DS[
5. Conclusion
In conclusion, the coherent DS intermittency is experimentally revealed in the net-normal-dispersion fiber laser. Different from conventional pulsating solitons and chaotic solitons, the intermittency manifests as robust switching between basic stages, maintaining coherence during evolution. At the low pump power, the intermittency manifests periodic switching between different pulsating solitons. The laser generates bi-stable DS with periodic wavelength switching by further increasing the pump power. The dominance of the slow-gain dynamics is indicated by the similarities between the random mode locking and the coherent DS intermittency. Future work will focus on finding a convincing explanation through numerical simulations, which needs to be implemented through a complete model containing the interaction between the broadband DS and gain medium. Our work offers novel insights into the nonlinear transient dynamics of the DS, promoting the understanding of ultrafast intermittency in fiber lasers.
References
[1] H. L. Chen, X. T. Jiang, S. X. Xu, H. Zhang. Recent progress in multi-wavelength fiber lasers: principles, status, and challenges. Chin. Opt. Lett., 18, 041405(2020).
[2] P. Grelu, N. Akhmediev. Dissipative solitons for mode-locked lasers. Nat. Photon., 6, 84(2012).
[3] A. Mahjoubfar, D. V. Churkin, S. Barland, N. Broderick, S. K. Turitsyn, B. Jalali. Time stretch and its applications. Nat. Photon., 11, 341(2017).
[4] P. Ryczkowski, M. Närhi, C. Billet, J. M. Merolla, G. Genty, J. M. Dudley. Real-time full-field characterization of transient dissipative soliton dynamics in a mode-locked laser. Nat. Photon., 12, 221(2018).
[5] G. Herink, B. Jalali, C. Ropers, D. R. Solli. Resolving the build-up of femtosecond mode-locking with single-shot spectroscopy at 90 MHz frame rate. Nat. Photon., 10, 321(2016).
[6] X. M. Liu, X.-K. Yao, Y.-D. Cui. Real-time observation of the buildup of soliton molecules. Phys. Rev. Lett., 121, 023905(2018).
[7] J. S. Peng, H. P. Zeng. Build-up of dissipative optical soliton molecules via diverse soliton interactions. Laser Photon. Rev., 12, 1800009(2018).
[8] J. M. Soto-Crespo, N. Akhmediev, A. Ankiewicz. Pulsating, creeping, and erupting solitons in dissipative systems. Phys. Rev. Lett., 85, 2937(2000).
[9] J. M. Soto-Crespo, M. Grapinet, P. Grelu, N. Akhmediev. Bifurcations and multiple-period soliton pulsations in a passively mode-locked fiber laser. Phys. Rev. E, 70, 066612(2004).
[10] C. Y. Bao, W. K. Chang, C. X. Yang, N. Akhmediev, S. T. Cundiff. Observation of coexisting dissipative solitons in a mode-locked fiber laser. Phys. Rev. Lett., 115, 253903(2015).
[11] Y. Q. Du, Z.-W. Xu, X.-W. Shu. Spatio-spectral dynamics of the pulsating dissipative solitons in a normal-dispersion fiber laser. Opt. Lett., 43, 3602(2018).
[12] J. S. Peng, S. Boscolo, Z. Zhao, H. P. Zeng. Breathing dissipative solitons in mode-locked fiber lasers. Sci. Adv., 5, eaax1110(2019).
[13] C. Chao, Q. Q. Huang, M. Al Araimi, A. Rozhin, S. Sergeyev, C. B. Mou. Observation of chaotic polarization attractors from a graphene mode locked soliton fiber laser. Chin. Opt. Lett., 17, 020012(2019).
[14] Z. Q. Wang, Q. Jiang, Z. Tang, Z. Zhang. Complex pulsating dynamics of counter-propagating solitons in a bidirectional ultrafast fiber laser. Opt. Express, 28, 28209(2020).
[15] J. Chen, X. Zhao, T. Li, J. J. Yang, J. S. Liu, Z. Zheng. Generation and observation of ultrafast spectro-temporal dynamics of different pulsating solitons from a fiber laser. Opt. Express, 28, 14127(2020).
[16] T. H. Xian, L. Zhan, W. C. Wang, W. Y. Zhang. Subharmonic entrainment breather solitons in ultrafast lasers. Phys. Rev. Lett., 125, 163901(2020).
[17] Y. Q. Du, M. M. Han, P. Y. Cheng, X. W. Shu. Pulsating soliton with broadened Kelly sidebands in an ultrafast fiber laser. Opt. Lett., 44, 4087(2019).
[18] M. Liu, Z. W. Wei, H. Li, T. J. Li, A. P. Luo, W. C. Xu, Z. C. Luo. Visualizing the “invisible” soliton pulsation in an ultrafast laser. Laser Photon. Rev., 14, 1900317(2020).
[19] S. H. Stogatz. Nonlinear Dynamicssa and Chaos(1994).
[20] S. T. Cundiff, J. M. Soto-Crespo, N. Akhmediev. Experimental evidence for soliton explosions. Phys. Rev. Lett., 88, 073903(2002).
[21] O. Descalzi, C. Cartes, J. Cisternas, H. R. Brand. Exploding dissipative solitons: the analog of the Ruelle–Takens route for spatially localized solutions. Phys. Rev. E, 83, 056214(2011).
[22] S. C. V. Latas, M. F. S. Ferreira. Why can soliton explosions be controlled by higher-order effects?. Opt. Lett., 36, 3085(2011).
[23] S. C. V. Latas, M. F. S. Ferreira. Soliton explosion control by higher-order effects. Opt. Lett., 35, 1771(2010).
[24] A. F. J. Runge, N. G. R. Broderick, M. Erkintalo. Observation of soliton explosions in a passively mode-locked fiber laser. Optica, 2, 36(2015).
[25] M. Liu, A. P. Luo, W. C. Xu, Z. C. Luo. Dissipative rogue waves induced by soliton explosions in an ultrafast fiber laser. Opt. Lett., 41, 3912(2016).
[26] Y. Q. Du, M.-M. Han, X.-W. Shu. Dark solitons in the exploding pulsation of the bright dissipative soliton in ultrafast fiber lasers. Opt. Lett., 45, 666(2020).
[27] F. C. Meng, C. Lapre, C. Billet, G. Genty, J. M. Dudley. Instabilities in a dissipative soliton-similariton laser using a scalar iterative map. Opt. Lett., 45, 1232(2020).
[28] Y. Q. Du, S. V. Sergeyev, Z. W. Xu, M. M. Han, X. W. Shu, S. K. Turitsyn. Alternation of the mode synchronization and desynchronization in ultrafast fiber laser. Laser Photon. Rev., 14, 1900219(2020).
[29] S. Lee, K. Park, H. Kim, L. A. Vazquez-Zuniga, J. Kim, Y. Jeong. Intermittent burst of a super rogue wave in the breathing multi-soliton regime of an anomalous fiber ring cavity. Opt. Express, 26, 11447(2018).
[30] M. Liu, T.-J. Li, A.-P. Luo, W.-C. Xu, Z.-C. Luo. “Periodic” soliton explosions in a dual-wavelength mode-locked Yb-doped fiber laser. Photon. Res., 8, 246(2020).
[31] C. R. Gile, S. E. Desurvire. Modeling erbium-doped fiber amplifier. IEEE J. Lightwavave Technol., 9, 271(1991).
[32] P. Sidorenko, W. Fu, F. W. Wise. Nonlinear ultrafast fiber amplifiers beyond the gain-narrowing limit. Optica, 6, 1328(2019).
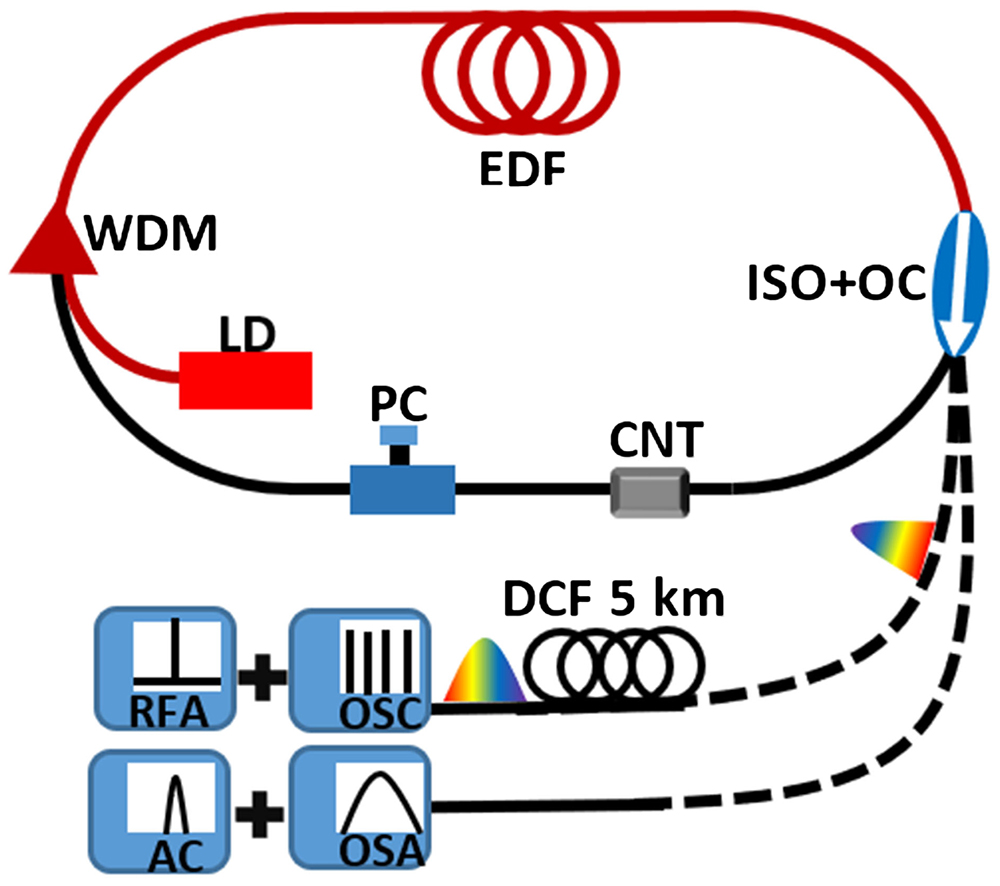
Set citation alerts for the article
Please enter your email address