
- Chinese Optics Letters
- Vol. 19, Issue 11, 110201 (2021)
Abstract
1. Introduction
The alignment and orientation of molecules have important and comprehensive application value in photoelectron angular distribution, chemical reaction dynamics, high-order harmonic generation, photoionization, photodissociation, and so on[
The ion-impact excitation of the diatomic molecule has been widely investigated in the absence of the laser field[
When exploring the molecular orientation, one often takes into account the influence of the external field. In the early days, the strong electrostatic field was usually used to control polar molecule orientation. The relevant experimental research showed that the hexapole electric field has advantages in orienting polarity symmetrical top or symmetrical top-like molecules[
Sign up for Chinese Optics Letters TOC. Get the latest issue of Chinese Optics Letters delivered right to you!Sign up now
The following explains how this Letter is arranged. Section 2 mainly outlines the density matrix theory method used in the calculation. In Section 3, we give the time evolution of the orientation degree in absence of an external field and how it is steered by laser pulses, and the influences of interaction potential and laser pulse parameters on molecular orientation degree are discussed and analyzed. Meanwhile, the influence of different temperatures on orientation is also compared. Finally, conclusions and perspectives are given in Section 4.
2. Theoretical Method
The molecular orientation was studied by considering the collision between an ion A and a diatomic molecule BC under a two-color laser pulse with STRT and a time-delayed THz laser pulse. The two-color laser pulse is expressed as
The interaction potential between the ion or proton A and molecule BC is described by[
Figure 1.Schematic diagram of the coordinate system formed by the collision of an ion or proton with a molecule.
In the eigenstates of the rigid rotor Hamiltonian, the density operator is written as
3. Results and Discussion
In this work, the parameters of the linear polarization molecule CO are as follows:
For clearly exhibiting the effect of the external fields on the collision-induced orientation, we first explore the time evolution of the molecular orientation under different conditions in Fig. 2. Figure 2(a) presents the orientation in the absence of an external field, and Fig. 2(b) shows the orientation degree in the THz field, the two-color field, and a combination of two laser pulses, respectively, where
Figure 2.Time evolution of the molecular orientation (a) in the absence of an external field and (b) being steered by laser pulses. Green line, THz laser pulse; orange line, two-color laser pulse; blue line, combination of two laser pulses. b = 10 a.u., v = 0.06 a.u., E01 = 5.1×107 V/cm, E02 = 1.0 × 107 V/cm, τ = 450 ps, ω1 = 12,500 cm−1, td = 2.16 ps, ωTHz = 36 cm−1, φ = π, and T = 0 K in all.
To display the variation of the maximal degree of orientation
Figure 3.Maximal degree of orientation as a function of the (a) collision velocity and (b) impact parameter.
To this end, we explore the impact of optimized two-color and THz field intensity on the molecular orientation induced by the
Figure 4.Maximal degree of orientation 〈cos θ〉max as a function of the field intensity of the (a) two-color and (b) THz field, where the b = 5 a.u., v = 0.03 a.u., and T = 0 K.
The CEP and frequency are significant parameters of THz laser pulse. Figure 5 shows the maximal degree of molecular orientation as a function of the CEP and frequency. As we can see from Fig. 5(a), by changing the THz laser pulse CEP from 0 to
Figure 5.Maximal degree of the orientation 〈cos θ〉max as a function of the (a) CEP and (b) frequency of the THz laser pulse, where b = 5 a.u., v = 0.03 a.u., E01 = 5.2 × 107 V/cm, E021 = 0.45 × 107 V/cm, and T = 0 K.
What was discussed above mainly concentrates on the effect of pulse and interaction parameters at temperature of 0 K. To further explore the influence of temperature on orientation degree, we changed the system temperature to investigate the degree of orientation induced by collision and modulated with combined laser pulses. Figure 6(a) gives the time evolution curves of molecular orientation under different temperatures. We can acquire the maximal orientation degrees of 0.825, 0.351, and 0.163 when the temperature
Figure 6.(a) Time evolution curves of orientation degree and (b) the rotational population of different rotational states at the temperature T = 0 K (blue line), 5 K (orange line), and 10 K (green line), while b = 5 a.u., v = 0.03 a.u., E01 = 5.2 × 107 V/cm, E02 = 0.45 × 107 V/cm, φ = 1.2π, and ωTHz = 26 cm-1.
4. Conclusion
In this work, the orientation of molecules induced by the
References
[1] L. Holmegaard, J. L. Hansen, L. Kalhøj, S. L. Kragh, H. Stapelfeldt, F. Filsinger, J. Kupper, G. Meijer, D. Dimitrovski, M. Abu-Samha, C. P. J. Martiny, L. B. Madsen. Photoelectron angular distributions from strong-field ionization of oriented molecules. Nat. Phys., 6, 428(2010).
[2] J. L. Hansen, H. Stapelfeldt, D. Dimitrovski, M. Abu-Samha, C. P. Martiny, L. B. Madsen. Time-resolved photoelectron angular distributions from strong-field ionization of rotating naphthalene molecules. Phys. Rev. Lett., 106, 073001(2011).
[3] J. Hu, M. S. Wang, K. L. Han, G. Z. He. Attosecond resolution quantum dynamics between electrons and H+2 molecules. Phys. Rev. A, 74, 063417(2006).
[4] J. Hu, K. L. Han, G. Z. He. Correlation quantum dynamics between an electron and D+2 molecule with attosecond resolution. Phys. Rev. Lett., 95, 123001(2005).
[5] T. Kanai, S. Minemoto, H. Sakai. Quantum interference during high-order harmonic generation from aligned molecules. Nature, 435, 470(2005).
[6] H. M. Wu, S. J. Yue, J. B. Li, S. L. Fu, B. Hu, H. C. Du. Controlling nonadiabatic spectral redshift of high-order harmonic using two orthogonally polarized laser fields. Chin. Opt. Lett., 16, 040203(2018).
[7] T. Kanai, S. Minemoto, H. Sakai. Ellipticity dependence of high-order harmonic generation from aligned molecules. Phys. Rev. Lett., 98, 053002(2007).
[8] D. Dimitrovski, J. Maurer, H. Stapelfeldt, L. B. Madsen. Observation of low-energy electrons in the photoelectron energy distribution from strong-field ionization of naphthalene by circularly polarized pulses. J. Phys. B, 48, 121001(2015).
[9] R. Siemering, O. Njoya, T. Weinacht, R. De. Vivie-Riedle. Field-dressed orbitals in strong-field molecular ionization. Phys. Rev. A, 92, 042515(2015).
[10] Z. Y. Zhao, Y. C. Han, J. Yu, S. L. Cong. The influence of field-free orientation on the predissociation dynamics of the NaI molecule. J. Chem. Phys., 140, 044316(2014).
[11] T. P. Rakitzis, A. J. van den Brom, M. H. M. Janssen. Directional dynamics in the photodissociation of oriented molecules. Science, 303, 1852(2004).
[12] J. Z. Shao, X. Liang, L. B. You, N. Pang, Y. Lin, S. M. Wang, Z. H. Deng, X. D. Fang, X. Wang. Laser-induced damage and periodic stripe structures of a CaF2 single crystal by an ArF excimer laser. Chin. Opt. Lett., 18, 021403(2020).
[13] Q. Guo, T. Liu, X. Q. Wang, Z. G. Zheng, A. Kudreyko, H. J. Zhao, V. Chigrinov, H. S. Kwok. Ferroelectric liquid crystals for fast switchable circular Dammann grating. Chin. Opt. Lett., 18, 080002(2020).
[14] L. B. Madsen, J. P. Hansen, J. Kocbach. Excitation in ion-atom collisions inside subfemtosecond laser pulses. Phys. Rev. Lett., 89, 093202(2002).
[15] V. Prasad, K. Yamashita. Effect of laser field ellipticity on the rovibrational excitations of a diatomic molecule. Chem. Phys. Lett., 426, 8(2006).
[16] Z. Z. Lu, D. Y. Chen, R. W. Fan, Y. Q. Xia. Laser-induced quadrupole-quadrupole collisional energy transfer in Xe-Kr systems. Phys. Rev. A, 85, 063402(2012).
[17] H. Y. Zhang, D. Y. Chen, Z. Z. Lu, R. W. Fan, Y. Q. Xia. Numerical calculation of laser-induced collisional energy transfer in Ba-Sr system. Acta Phys. Sin., 57, 7600(2008).
[18] G. Paterson, A. Relf, M. L. Costen, K. G. McKendrick, M. H. Alexander, P. J. Dagdigian. Rotationally elastic and inelastic dynamics of NO(X2Π, v = 0) in collisions with Ar. J. Chem. Phys., 135, 234304(2011).
[19] M. Brouard, S. D. S. Gordon, A. Hackett Boyle, C. G. Heid, B. Nichols, V. Walpole, F. J. Aoiz, S. Stolte. Integral steric asymmetry in the inelastic scattering of NO(X2Π). J. Chem. Phys., 146, 014302(2017).
[20] R. D. Levine, R. B. Bernstein. Molecular Reaction Dynamics and Chemical Reactivity(1987).
[21] M. Mohan, V. Prasad. Laser-assisted vibrational excitations during ion-molecule collisions. J. Phys. B, 24, L81(1991).
[22] M. C. van Beek, J. J. ter Meulen, M. H. Alexander. Rotationally inelastic collisions of OH(X2Π) + Ar. I. State-to-state cross sections. J. Chem. Phys., 113, 628(2000).
[23] M. Brouard, H. Chadwick, S. D. S. Gordon, B. Hornung, B. Nichols, F. J. Aoiz, S. Stolte. Rotational orientation effects in NO(X) + Ar inelastic collisions. J. Phys. Chem. A, 119, 12404(2015).
[24] D. Zanuttini, I. Blum, E. di Russo, L. Rigutti, F. Vurpillot, J. Douady, E. Jacquet, P.-M. Anglade, B. Gervais. Dissociation of GaN2+ and AlN2+ in APT: analysis of experimental measurements. J. Chem. Phys., 149, 134311(2018).
[25] E. Di Russo, I. Blum, I. Rivalta, J. Houard, G. Da Costa, F. Vurpillot, D. Blavette, L. Rigutti. Detecting dissociation dynamics of phosphorus molecular ions by atom probe tomography. J. Phys. Chem. A, 124, 10977(2020).
[26] R. B. Bernstein. Analysis of the experimental orientation dependence of the reaction CH3I + Rb→RbI +CH3. J. Chem. Phys., 82, 3656(1985).
[27] B. Friedrich, D. Herschbach. Enhanced orientation of polar molecules by combined electrostatic and nonresonant induced dipole forces. J. Chem. Phys., 111, 6157(1999).
[28] L. Cai, J. Marango, B. Friedrich. Time-dependent alignment and orientation of molecules in combined electrostatic and pulsed nonresonant laser fields. Phys. Rev. Lett., 86, 775(2001).
[29] S. A. Zhang, C. H. Lu, T. Q. Jia, Z. G. Wang, Z. R. Sun. Field-free molecular orientation enhanced by two dual-color laser subpulses. J. Chem. Phys., 135, 034301(2011).
[30] J. Wu, H. P. Zeng. Field-free molecular orientation control by two ultrashort dual-color laser pulses. Phys. Rev. A, 81, 053401(2010).
[31] S. A. Zhang, J. H. Shi, H. Zhang, T. Q. Jia, Z. G. Wang, Z. R. Sun. Field-free molecular orientation by a multicolor laser field. Phys. Rev. A, 83, 023416(2011).
[32] C. C. Shu, K. J. Yuan, W. H. Hu, J. Yang, S. L. Cong. Controlling the orientation of polar molecules in a rovibrationally selective manner with an infrared laser pulse and a delayed half-cycle pulse. Phys. Rev. A, 78, 055401(2008).
[33] Q. Y. Cheng, J. S. Liu, X. C. Zhou, Y. Z. Song, Q. T. Meng. Field-free alignment dynamics of FCN molecule induced by a terahertz half-cycle pulse. Europhys. Lett., 125, 33001(2019).
[34] M. Muramatsu, M. Hita, S. Minemoto, H. Sakai. Field-free molecular orientation by an intense nonresonant two-color laser field with a slow turn on and rapid turn off. Phys. Rev. A, 79, 011403(2009).
[35] J. S. Liu, Q. Y. Cheng, D. G. Yue, X. C. Zhou, Q. T. Meng. Dynamical analysis of the effect of elliptically polarized laser pulses on molecular alignment and orientation. Chin. Opt. Lett., 16, 103201(2018).
[36] Y. Liu, J. Li, J. Yu, S. L. Cong. Field-free molecular orientation by two-color shaped laser pulse with time-delayed THz laser pulse. Laser Phys. Lett., 10, 076001(2013).
[37] J. S. Liu, Q. Y. Cheng, D. G. Yue, X. C. Zhou, Q. T. Meng. Influence factor analysis of field-free molecular orientation. Chin. Phys. B, 27, 033301(2018).
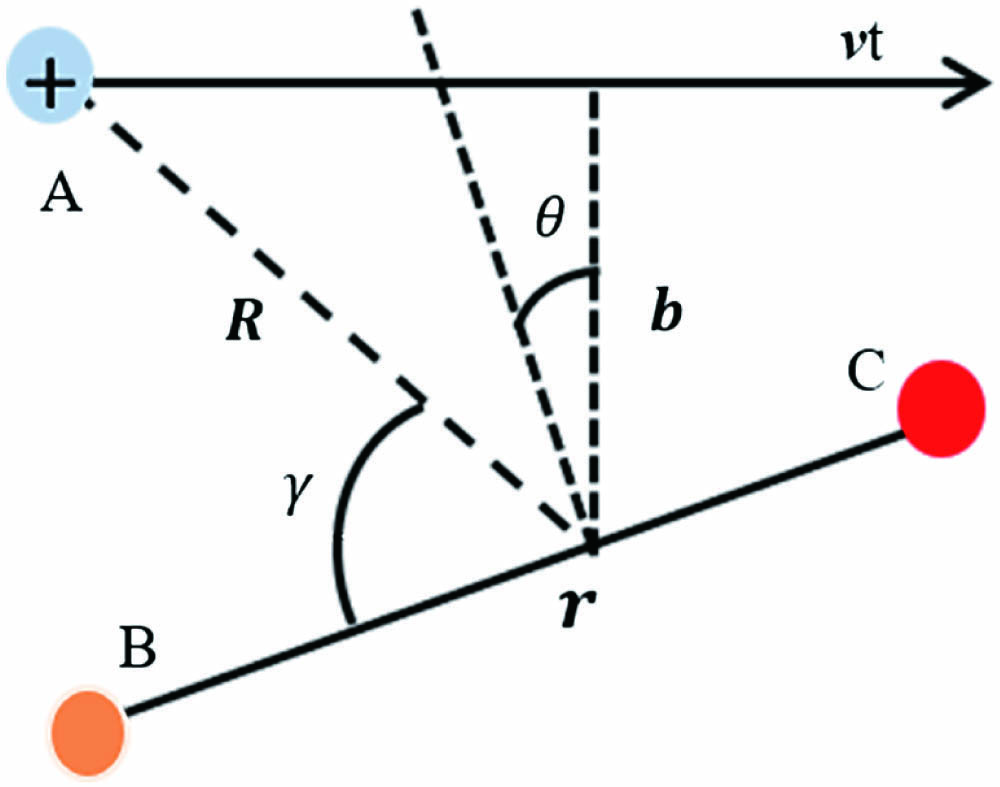
Set citation alerts for the article
Please enter your email address