W. L. Zhang*, Y. B. Song, X. P. Zeng, R. Ma, Z. J. Yang, and Y. J. Rao
Abstract
In this paper, we proposed a way to realize an Er-doped random fiber laser (RFL) with a disordered fiber Bragg grating (FBG) array, as well as to control the lasing mode of the RFL by heating specific locations of the disordered FBG array. The disordered FBG array performs as both the gain medium and random distributed reflectors, which together with a tunable point reflector form the RFL. Coherent multi-mode random lasing is obtained with a threshold of between 7.5 and 10 mW and a power efficiency between 23% and 27% when the reflectivity of the point reflector changes from 4% to 50%. To control the lasing mode of random emission, a specific point of the disordered FBG array is heated so as to shift the wavelength of the FBG(s) at this point away from the other FBGs. Thus, different resonance cavities are formed, and the lasing mode can be controlled by changing the location of the heating point.1. INTRODUCTION
Random lasers (RLs) are disordered optical structures of stimulated emission in which light waves are both multiply scattered and amplified. Fabrication of RLs is simple and cost effective (i.e., no fine mirrors or a well-defined cavity are needed), especially for nanoscale structures. RLs have the characteristics of low spacial coherence and wide emission angle, while keeping a high -factor and high photon density of state that is comparable to those of ordinary lasers [1–3]. Thus, RLs are attractive for both fundamental theoretical and technological research and have great potential applications in wide areas such as photonic information, imaging, and display.
One of the main challenges in developing RLs is a lack of ways to regulate their output (e.g., laser mode selection and modulation), because the randomly formed feedback loops of light are difficult to specify or access. A few groups have attempted to tune and control RLs in different ways, such as temperature tuning, resonance driving, variation of effective lifetime of the cavity, and choice of different pump beam profiles [4–6]. Recent achievements of random fiber lasers (RFLs) in low dimensional waveguides also provide fine ways to tailor their output [7–12]. Some inherent characteristics of fiber waveguides (e.g., slow light, tight transverse mode confinement, and rich interaction of inner fields) offer efficient ways to control laser output.
As one of the three typical types of RFLs [13], an active fiber with random mirrors of distributed or phase-shifted Bragg gratings [14–17] emits coherent light with a low lasing threshold compared with the other two types. However, such RFLs exhibit several coherent random lasing modes, and the output is less stable because of mode competition or cavity thermal effect. Hence, it is important to stabilize or even to select the emission mode of these RFLs, which is of great significance to realize high-efficiency, mode/wavelength controllable, and dynamically tunable RFLs.
Sign up for Photonics Research TOC. Get the latest issue of Photonics Research delivered right to you!Sign up now
In RFLs, passive methods such as introducing wavelength tunable components and mixing different types of fibers have been proposed to optimize the output characteristics. However, these methods have less flexibility of light control. Temperature tuning, spatial phase modulation [4–6], and so forth have been demonstrated for actively controlling random lasing in bulk structures. However, these active methods of RL control have been studied less in RFLs. In one of our recent works, an optical control method (i.e., introducing control light laterally as spatial gain perturbation) of an Er-doped RFL was proposed to select individual modes of random emission [13]. We propose a new way to control lasing modes of an Er-doped RFL by heating different positions of the disordered fiber Bragg grating (FBG) array. Besides, a tunable point reflector is introduced to optimize output characteristics of the RFLs. The experimental results show that several stable lasing modes can be selected by heating different positions of the disordered FBG array. Compared with the method of light control, the thermal control is simpler, more cost efficient, and has a relatively wider heating range (e.g., a large area and high power density light beam is difficult to obtain). The proposed method provides a dynamic and flexible means of laser mode control in RFLs. The location dependent mode selection mechanism could also be a potential means for laser mode switching in applications for information optics.
2. PRINCIPLE OF OPERATION
A schematic diagram of the experimental setup is shown in Fig. 1. A 1480 nm laser is used as the pump laser, whose output is coupled into the gain fiber through a 1480/1550 nm wavelength division multiplexer (WDM). The gain fiber is composed of a 7 m length erbium-doped fiber (EDF), with an array of 20 FBGs distributed randomly at the end of the EDF. Each of the FBGs has a reflectivity of about 4% and a central wavelength at about 1552.5 nm. The length of each FBG is 3 mm, while the separation between two neighboring FBGs is chosen randomly in the range of 2–8 mm. A detailed description of the disordered FBG array and the EDF is given in [13,17]. The 1550 nm port of the WDM is connected to a 1:99 coupler. A fiber loop mirror (formed by a 1:1 coupler) and a variable attenuator are used together as the tunable point reflector, whose reflectivity can be adjusted by changing the attenuation of the variable attenuator. Output of the RFL is monitored at Port A through a power meter or an optical spectrum analyzer (OSA).
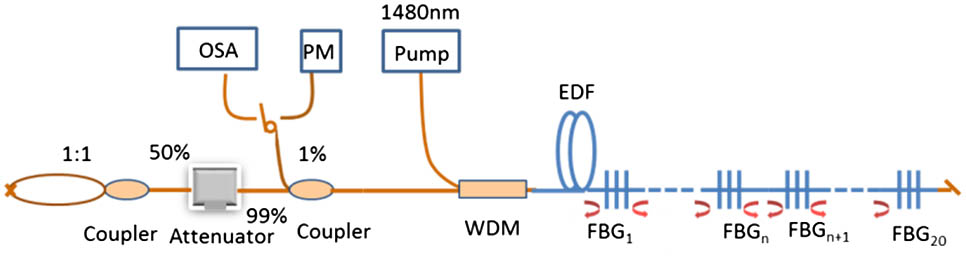
Figure 1.Schematic diagram of the experimental setup of the RFL. PM, power meter.
Through this configuration, the tunable point reflector can form a resonance cavity with any one of the randomly distributed FBGs. Moreover, conditions of light localization are also satisfied between the FBGs (i.e., the localization length of the disordered FBG array is shorter than the separation between the FBGs [13,18]). Thus, random lasing modes in the coherent regime can be obtained when gain overcomes loss.
3. RESULTS AND ANALYSIS
A. Slope Efficiency at Different Reflectivity
First, we studied the lasing threshold and efficiency of the RFL for different values of reflectivity of the tunable reflector, or rt. Figure 2 gives the output–input curve of the proposed RFL. When rt changes from 4% to 50%, the lasing efficiency (i.e., optical to optical) increases from 23% to 27%, and the lasing threshold decreases from 10 to 7.5 mW. We mainly consider the condition when and . corresponds to the case of Fresnel reflection, which can be realized by cutting the fiber end smoothly. corresponds to the case when a loop mirror is used. From our study, the output spectra become more stable, and the emission efficiency increases when rt increases. To this end is preferred. It is easy to understand that a higher value of rt is helpful to reduce the lasing threshold as well as to increase the lasing efficiency of the RFL, because resonance modes with higher quality factors will be formed. As we can see from Fig. 2, the change of laser threshold and slope efficiency is relatively small. When the reflectivity increases, the output power does not change much from this port, since most of the power is reflected to the right direction.
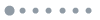
Figure 2.Output power versus pump power for rt of 4%, 25%, and 50%, respectively.
B. Spectra of Different Reflectivity
Correspondingly, the lasing spectrum of the RFL for a fixed value of pump power is shown in Fig. 3. When , as seen in Figs. 3(a)–3(c), power distribution of the output spectrum fluctuates randomly with time. Multiple peaks appear randomly in the laser spectrum, corresponding to emission of different localized modes in the one-dimensional (1D) disordered structure formed by the FBG arrays. When rt increases to 50%, the output becomes quasi-stable. For example, only one peak appears in the laser spectrum, and the shape of the spectrum keeps almost unchanged with time; see Figs. 3(d)–3(f).
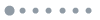
Figure 3.Output spectra of the RFL measured at different times; (a)–(c) correspond to , (d)–(f) correspond to . The pump power is 67.7 mW.
C. Influence of Temperature
To control the lasing mode of random emission, a specific point of the disordered FBG array is heated so as to shift the wavelength of the FBG(s) at this point away from the other FBGs. In this way, the 1D disordered structure is adjusted so different modes of light localization will be formed, giving birth to random lasing modes that are dependent on the position of the heating point. In our experiment, an electric iron is used as the heater, which is set with a fixed distance to specific heating points of the fiber.
Figures 4 and 5 show the selected lasing mode when different positions of the fiber are heated, for pump power of 67.7 mW and rt equal to 4% and 50%, respectively. We only considered the cases when a stable single lasing mode is selected. As illustrated in Fig. 4, single mode emission with 3 dB linewidth of about 0.01 nm and peak wavelength at 1552.448, 1552.546, 1552.490, 1552.496, 1552.522, and 1552.528 nm are obtained when the heating position is set at 7.5, 11.2, 11.5, 12, 12.5, and 14.5 cm, respectively. It is observed that some unexcited modes in Figs. 3(a)–3(c) can be selected to emit when heating specific points of the fiber. This indicates that the light localization changes correspondingly with the adjustment of the disordered structure; thus, position-dependent lasing modes can be selected.
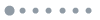
Figure 4.Output spectra of the stable and single mode emission when heating different locations of the FBG array. In the experiment, and the pump power is 67.7 mW.
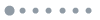
Figure 5.Output spectra of the stable and single mode emission when heating different locations of the FBG array. In the experiment, and the pump power is 67.7 mW.
It is worth noting that the selected mode is much more stable compared with the free running RFL (i.e., when no point of the fiber is heated). Figure 6 shows time variation of the peak power and peak wavelength of the lasing spectrum. It is observed that fluctuations of the peak wavelength and the peak power are less than 0.01% and 10%, respectively. Figure 7 shows variation of the peak power and peak wavelength of the lasing spectrum in 1 h. We measure the spectra every 3 min for 1 h and obtain 20 data points. As we can see from Fig. 7, the single mode lasing is stable, the peak power here nearly stays unchanged, and the fluctuation of peak wavelength is less than 0.01%.
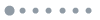
Figure 6.Peak wavelength and peak power of the selected modes versus time. In the experiment, and the pump power is 67.7 mW.
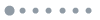
Figure 7.Peak wavelength and peak power of the selected modes versus time. In the experiment, and the pump power is 67.7 mW.
Figure 8 shows the peak wavelength of the selected modes as a function of the heating location. For and 50%, the two curves vary randomly versus heating location; however, they show similar fluctuations as a function of the heating location. This verifies that the lasing wavelength is related with the localized mode corresponding to disordered distribution of the FBGs.
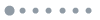
Figure 8.Peak wavelength of the stable and single mode emission versus control location for rt of 4% and 50%, respectively.
4. CONCLUSION
In summary, we have realized an effective method to control the lasing mode of an Er-doped RFL, wherein a disordered FBG array is used to provide gain and random feedback. The RFL exhibits multi-mode random lasing or single mode lasing depending on the reflectivity of the additional point reflectors. By heating specific positions of the FBG array, stable single mode lasing can be obtained. Moreover, different lasing modes can be selected through changing the heating point that adjusts light localization in the disordered FBG array. This study provides an efficient method for mode stabilization and selection of similar types of RFLs. The spatial-dependent characteristic of mode selection also provides a potential means of laser mode encoding/switching for optical privacy communication or multiplexing. For this kind of laser, this is the first time a thermal control method has been used to select random lasing modes and realize single mode lasing. Thermal control provides a wider heating range (or multiple heating points), which might be used in subsequent studies for complex mode selection of the RFL, for instance, selecting multiple random lasing modes simultaneously or selecting a random lasing mode that cannot be excited when only one heating point is used.
Acknowledgment
Acknowledgment. The authors would like to thank Professor Adrian Podoleanu at University of Kent and Professor Sergei K. Turitsyn at Aston University for their useful discussions. This work was supported in part by the National Natural Science Foundation of China under Grants 61575040 and 61106045, the PCSIRT under Grant IRT1218, the 111 Project under Grant B14039, and the open research fund of Jiangsu Key Laboratory for Advanced Optical Manufacturing Technologies under Grant KJS1402.
References
[1] D. S. Wiersma. The physics and applications of random lasers. Nat. Phys., 4, 359-367(2008).
[2] J. Andreasen, A. A. Asatryan, L. C. Botten, M. A. Byrne, H. Cao, L. Ge, L. Labonté, P. Sebbah, A. D. Stone, H. E. Türeci, C. Vanneste. Modes of random lasers. Adv. Opt. Photon., 3, 88-127(2010).
[3] J. Liu, P. D. García, S. Ek, N. Gregersen, T. Suhr, M. Schubert, J. Mørk, S. Stobbe, P. Lodahl. Random nanolasing in the Anderson localized regime. Nat. Nanotechnol., 9, 285-289(2014).
[4] S. Gottardo, R. Sapienza, P. D. Garcia, A. Blanco, D. S. Wiersma, C. Lopez. Resonance-driven random lasing. Nat. Photonics, 2, 429-432(2008).
[5] M. Leonetti, C. Conti, C. Lopez. The mode-locking transition of random lasers. Nat. Photonics, 5, 615-617(2011).
[6] N. Bachelard, S. Gigan, X. Noblin, P. Sebbah. Adaptive pumping for spectral control of random lasers. Nat. Phys., 10, 426-431(2014).
[7] S. Turitsyn, S. Babin, D. Churkin, I. Vatnik, M. Nikulin, E. Podivilov. Random distributed feedback fibre lasers. Phys. Rep., 542, 133-193(2014).
[8] D. V. Churkin, S. A. Babin, A. E. El-Taher, P. Harper, S. I. Kablukov, V. Karalekas, J. D. Ania-Castanon, E. V. Podivilov, S. K. Turitsyn. Raman fiber lasers with a random distributed feedback based on Rayleigh scattering. Phys. Rev. A, 82, 003828(2010).
[9] W. L. Zhang, Y. J. Rao, Z. X. Yang, Z. N. Wang, X. H. Jia. Low threshold 2nd-order random lasing of a fiber laser with a half-opened cavity. Opt. Express, 20, 14400-14405(2012).
[10] H. Zhang, P. Zhou, H. Xiao, X. Xu. Efficient Raman fiber laser based on random Rayleigh distributed feedback with record high power. Laser Phys. Lett., 11, 075104(2014).
[11] S. A. Babin, I. D. Vatnik, A. Y. Laptev, M. M. Bubnov, E. M. Dianov. High-efficiency cascaded Raman fiber laser with random distributed feedback. Opt. Express, 22, 24929-24934(2014).
[12] Z. Hu, Q. Zhang, B. Miao, Q. Fu, G. Zou, Y. Chen, Y. Luo, D. Zhang, P. Wang, H. Ming, Q. Zhang. Coherent random fiber laser based on nanoparticles scattering in the extremely weakly scattering regime. Phys. Rev. Lett., 109, 253901(2012).
[13] W. L. Zhang, R. Ma, C. H. Tang, Y. J. Rao, X. P. Zeng, Z. J. Yang, Z. N. Wang, Y. Gong, Y. S. Wang. All optical mode controllable Er-doped random fiber laser with distributed Bragg gratings. Opt. Lett., 40, 3181-3184(2015).
[14] N. Lizárraga, N. P. Puente, E. I. Chaikina, T. A. Leskova, E. R. Méndez. Single-mode Er-doped fiber random laser with distributed Bragg grating feedback. Opt. Express, 17, 395-404(2009).
[15] M. Gagné, R. Kashyap. Demonstration of a 3 mW threshold Er-doped random fiber laser based on a unique fiber Bragg grating. Opt. Express, 17, 19067-19074(2009).
[16] L. Wang, X. Dong, P. P. Shum, X. Liu, H. Su. Random laser with multiphase-shifted Bragg grating in Er/Yb-codoped fiber. J. Lightwave Technol., 33, 95-99(2015).
[17] W. L. Zhang, S. W. Li, R. Ma, Y. J. Rao, Y. Y. Zhu, Z. N. Wang, X. H. Jia, J. Li. Random distributed feedback fiber laser based on combination of Er-doped fiber and single-mode fiber. IEEE J. Sel. Top. Quantum Electron., 21, 44-49(2015).
[18] V. Milner, A. Z. Genack. Photon localization laser: low-threshold lasing in a random amplifying layered medium via wave localization. Phys. Rev. Lett., 94, 073901(2005).