
- Chinese Optics Letters
- Vol. 19, Issue 11, 111901 (2021)
Abstract
Keywords
1. Introduction
Coherent data modulation and detection enable high capacity and spectral efficiency optical transmissions by encoding multi-level quadrature information onto both the amplitude and phase of a laser carrier. At the receiver, the arrived coherent data signal is demodulated by mixing itself with a local oscillator (LO)[
A potential solution is known as the self-homodyne detection (SHD) technique[
The performance metrics of all-optical carrier recovery include the following parameters. First, the spectral bandwidth for carrier extraction needs to be as small as possible, so that the data component can be located as close as possible to the carrier tone, thus maximizing the spectrum utilization. Second, the optical signal-to-noise ratio (OSNR) of the recovered carrier should be as high as possible (namely, the spectral component of the data signal should be excluded as clear as possible), so that residual high-frequency noise attached to the LO tone is avoided. Third, it will be beneficial if the carrier tone can be amplified while being extracted from the data signal. To this extent, carrier recovery methods implemented via nonlinear effects such as SBS[
Sign up for Chinese Optics Letters TOC. Get the latest issue of Chinese Optics Letters delivered right to you!Sign up now
As demonstrated in our recent work[
Figure 1.Comparison of all-optical carrier recovery using (a) free-running and (b) injection locked Brillouin laser in optical microcavity.
More recently, it has been reported that Brillouin lasing dynamics in a silicon opto-mechanics resonator and silica spherical microcavity can be injection-locked by launching a small seed laser within the Brillouin gain spectrum[
Here, we demonstrate comprehensive investigation of the injection locking dynamics of a backscattered Brillouin laser in a silica WGM microcavity[
2. Experiments and Discussions
The experimental setup is shown in Fig. 2(a). We adopt a silica WGM micro-rod cavity as the Brillouin laser platform. The WGM cavity is fabricated by
Sign up for Chinese Optics Letters TOC. Get the latest issue of Chinese Optics Letters delivered right to you!Sign up now
Figure 2.Demonstration of injection locking of a Brillouin laser in a WGM microcavity. (a) Experimental setup of our study. The inset shows the optical microscopic photo of the silica micro-rod cavity used in our experiment. (b) Ring down measurement of a microcavity mode exhibiting a Q-factor of 2.6 billion. (c) Optical spectra of the Brillouin laser and the AM sideband used as the seed laser. (d) Comparison of the beat note between the generated Brillouin laser and the pump laser with or without injection locking. The inset shows the close-up beat note spectrum at −10 dB injection ratio. (e) Injection locking range of Brillouin laser under different injection ratios.
Then, we modulated the pump laser using a Mach–Zehnder modulator (MZM) to generate a 11.11 GHz amplitude modulation (AM) sideband as the seed laser tone and send it into the microcavity from the backward direction (i.e., the same direction as the Brillouin laser, opposite direction to the pump laser). The seed laser power is set to be 10–20 dB lower than the pump laser power (i.e., the injection ratio ranges from
Next, we explore the injection locked Brillouin laser to facilitate all-optical carrier recovery in the scenario of coherent optical communication. As illustrated in Fig. 3(a), first, an in-phase and quadrature (IQ) modulator is utilized to generate 18.0 Gbaud 16-quadrature amplitude modulation (QAM) optical orthogonal frequency division multiplexing (OFDM) data signal[
Figure 3.Demonstration of all-optical recovery and SHD data receiving using injection locked Brillouin laser. (a) Experimental setup. (b) SNR, constellation map, and BER measurement of the received 16-QAM OFDM data signal. (c) Phase drifts between the data signal and LO using injection locked and free-running Brillouin lasers.
The Brillouin laser is then coupled out from the microcavity and sent into the IQ receiver as the LO for coherent SHD receiving. At the input of the coherent receiver, the data signal power is set to
3. Conclusion
We have demonstrated injection locking of backscattered Brillouin laser in a silica WGM microcavity. It has been observed that the frequency and phase of the seed laser can be faithfully imposed to the Brillouin laser within an injection range of 486 kHz (213 kHz) at the injection ratio of
References
[1] P. J. Winzer, D. T. Neilson, A. R. Chraplyvy. Fiber-optic transmission and networking: the previous 20 and the next 20 years. Opt. Express, 26, 24190(2018).
[2] E. Ip, A. P. T. Lau, D. J. F. Barros, J. M. Kahn. Coherent detection in optical fiber systems. Opt. Express, 16, 753(2008).
[3] X. Liu, S. Chandrasekhar, P. J. Winzer. Digital signal processing techniques enabling multi-Tb/s superchannel transmission: an overview of recent advances in DSP-enabled superchannels. IEEE Signal Process. Mag., 31, 16(2014).
[4] C. Laperle, M. O’Sullivan. Advances in high-speed DACs, ADCs, and DSP for optical coherent transceivers. J. Lightwave Technol., 32, 629(2014).
[5] B. J. Puttnam, R. S. Luis, J. M. Delgado Mendinueta, J. Sakaguchi, W. Klaus, Y. Kamio, M. Nakamura, N. Wada, Y. Awaji, A. Kanno, T. Kawanishi, T. Miyazaki. Self-homodyne detection in optical communication systems. Photonics, 1, 110(2014).
[6] B. J. Puttnam, R. Luis, J.-M. Delgado-Mendinueta, J. Sakaguchi, W. Klaus, Y. Awaji, N. Wada, A. Kanno, T. Kawanishi. High-capacity self-homodyne PDM-WDM-SDM transmission in a 19-core fiber. Opt. Express, 22, 21185(2014).
[7] M. Mazur, A. Lorences-Riesgo, J. Schroder, P. A. Andrekson, M. Karlsson. 10 Tb/s PM-64QAM self-homodyne comb-based superchannel transmission with 4% shared pilot tone overhead. J. Lightwave Technol., 36, 3176(2018).
[8] S. Adhikari, S. L. Jansen, M. Alfiad, B. Inan, A. Lobato, V. A. J. M. Sleiffer, W. Rosenkranz. Experimental investigation of self coherent optical OFDM systems using Fabry–Perot filters for carrier extraction. 36th European Conference and Exhibition on Optical Communication, 1(2010).
[9] Z. Liu, J.-Y. Kim, D. J. Richardson, R. Slavík. Homodyne OFDM with optical injection locking for carrier recovery. J. Lightwave Technol., 33, 34(2015).
[10] E. Giacoumidis, A. Choudhary, E. Magi, D. Marpaung, K. Vu, P. Ma, D. Y. Choi, S. Madden, B. Corcoran, M. Pelusi, B. J. Eggleton. Chip-based Brillouin processing for carrier recovery in self-coherent optical communications. Optica, 5, 1191(2018).
[11] M. Pelusi, A. Choudhary, T. Inoue, D. Marpaung, B. J. Eggleton, K. S. Trapala, H. N. Tan, S. Namiki. Frequency comb noise suppressor by a Brillouin comb amplifier for phase sensitive communications. Opt. Express, 25, 17847(2017).
[12] Q. Wen, J. Qin, Y. Geng, G. Deng, Q. Zhou, H. Zhou, K. Qiu. Stimulated Brillouin laser-based carrier recovery in a high-Q microcavity for coherent detection. Opt. Lett., 45, 3848(2020).
[13] Y. Geng, W. Cui, Q. Wen, B. Wang, Q. Zhou, B. Wu, H. Zhou. Microcavity-based narrowband parametric amplifier for carrier recovery in optical coherent self-homodyne detection. Opt. Lett., 44, 3490(2019).
[14] A. Debut, S. Randoux, J. Zemmouri. Linewidth narrowing in Brillouin lasers: theoretical analysis. Phys. Rev. A, 62, 023803(2000).
[15] W. Loh, S. B. Papp, S. A. Diddams. Noise and dynamics of stimulated-Brillouin-scattering microresonator lasers. Phys. Rev. A, 91, 053843(2015).
[16] S. Gundavarapu, G. M. Brodnik, M. Puckett, T. Huffman, D. Bose, R. Behunin, J. Wu, T. Qiu, C. Pinho, N. Chauhan, J. Nohava, P. T. Rakich, K. D. Nelson, M. Salit, D. J. Blumenthal. Sub-hertz fundamental linewidth photonic integrated Brillouin laser. Nat. Photon., 13, 60(2019).
[17] N. T. Otterstrom, R. O. Behunin, E. A. Kittlaus, Z. Wang, P. T. Rakich. A silicon Brillouin laser. Science, 360, 1113(2018).
[18] L. Sheng, D. Ba, Z. Lü. Weak laser pulse signal amplification based on a fiber Brillouin amplifier. Chin. Opt. Lett., 16, 111901(2018).
[19] Y. Bai, M. Zhang, Q. Shi, S. Ding, Y. Qin, Z. Xie, X. Jiang, M. Xiao. Brillouin–Kerr soliton frequency combs in an optical microresonator. Phys. Rev. Lett., 126, 063901(2021).
[20] N. T. Otterstrom, S. Gertler, Y. Zhou, E. A. Kittlaus, R. O. Behunin, M. Gehl, A. L. Starbuck, C. M. Dallo, A. T. Pomerene, D. C. Trotter, A. L. Lentine, P. T. Rakich. Backscatter-immune injection-locked Brillouin laser in silicon. Phys. Rev. A, 14, 044042(2020).
[21] F. Zhang, Y. Feng, X. Chen, L. Ge, W. Wan. Synthetic anti-PT symmetry in a single microcavity. Phys. Rev. Lett., 124, 053901(2020).
[22] P. Del’Haye, S. A. Diddams, S. B. Papp. Laser-machined ultra-high-Q microrod resonators for nonlinear optics. Appl. Phys. Lett., 102, 221119(2013).
[23] Q. Wen, W. Cui, Y. Geng, H. Zhou, K. Qiu. Precise control of micro-rod resonator free spectral range via iterative laser annealing. Chin. Opt. Lett., 19, 071903(2021).
[24] D. A. Korobko, I. O. Zolotovskii, V. V. Svetukhin, A. V. Zhukov, A. N. Fomin, C. V. Borisova, A. A. Fotiadi. Detuning effects in Brillouin ring microresonator laser. Opt. Express, 28, 4962(2020).
[25] Z. Liu, R. Slavík. Optical injection locking: from principle to applications. J. Lightwave Technol., 38, 43(2020).
[26] W. Loh, A. A. S. Green, F. N. Baynes, D. C. Cole, F. J. Quinlan, H. Lee, K. J. Vahala, S. B. Papp, S. A. Diddams. Dual-microcavity narrow-linewidth Brillouin laser. Optica, 2, 225(2015).
[27] X. Yi, W. Shieh, Y. Tang. Phase estimation for coherent optical OFDM. IEEE Photon. Technol. Lett., 19, 919(2007).
[28] X. Fang, F. Zhang. Phase noise estimation and suppression for PDM CO-OFDM/OQAM systems. J. Lightwave Technol., 35, 1837(2017).
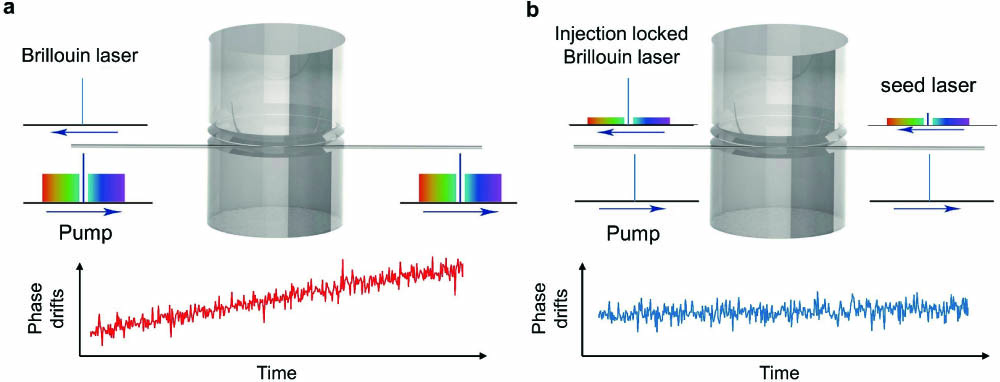
Set citation alerts for the article
Please enter your email address