Abstract
Transverse mode characteristics of a laser are related to a variety of interesting applications. An on-demand mode solid laser in the 1064 nm band was proposed previously. In this paper, we provide a fiber laser for on-demand modes in the 1550 nm band to prescribe the pure and high-quality emission of a higher-order transverse laser mode, based on a simple construction with one spatial light modulator (SLM) and a single-mode erbium-doped fiber (SM-EDF). The SLM is designated to generate the desired higher-order mode and separate the higher-order mode and the fundamental mode. The fundamental mode oscillates in the fiber ring laser, and therefore the SM-EDF can be pumped with a single-mode 980 nm laser, no matter what higher-order mode is prescribed. In this proof-of-principle experiment, high-quality higher-order modes are observed from to . Stable emission and real-time switching between modes can be easily realized by altering the phase on the SLM. In addition, the propagation of the , , , and modes from the fiber laser is also demonstrated in a four-mode few-mode fiber.1. INTRODUCTION
Transverse mode characteristics of lasers have been extensively investigated with a wide range of applications, such as space-division multiplexing (SDM) optical fiber communications [1–3], optical fiber sensing [4,5], quantum information [6,7], and laser processing of materials [8]. With the purpose of improving the transmission capacity in optical communication, the information is modulated at several different higher-order modes [9–11]. In an optical fiber sensor, the higher-order modes can achieve high discrimination accuracy for temperature and strain [12]. In quantum information technologies [13], the higher-order mode at the single photon level has also been exploited for entanglement experiments, quantum cryptography, and high-density information transfer. In laser processing of materials, the higher-order modes can be employed for laser ablation [8].
For specific applications, accurate and pure transverse mode emission is commonly required. In a conventional laser, the laser oscillates with both the higher-order modes and the fundamental mode [14]. Consequently, it is difficult to customize and prescribe the pure higher-order mode according to the specific application. Recently, attention has been focused on the on-demand mode laser that oscillates in a single transverse higher-order mode, which is a straightforward and efficient way to generate the specific mode directly.
So far, an on-demand mode solid-state laser has been proposed in the 1064 nm band [15]. In this scheme, the flexibility of the spatial light modulator (SLM) is utilized to customize and prescribe the pure higher-order mode. In this solid-state laser, an Nd:YAG crystal is employed and end pumped with a multi-mode fiber-coupled laser diode operating at 808 nm. The output mode can be customized and switched by controlling the SLM. This method can generate an accurate and pure transverse mode in the 1064 nm band. However, it is difficult to extend to the 1550 nm band, which is the window of lowest loss for the optical fiber. Therefore, there is an urgent need to realize the on-demand mode in the 1550 nm band. In 1550 nm band, the most frequently used crystal is an Er:glass crystal, whose emission cross section is approximately 3 orders of magnitude less than that of the Nd:YAG operating in the 1064 nm band. As a result, the Er:glass crystal can be used as the gain medium to generate the fundamental mode, despite the fact that it cannot excite the higher-order modes due to insufficient gain. To be specific, in the method proposed in Ref. [15], it is difficult to prescribe the higher-order transverse mode in the 1550 nm band. Accordingly, it is necessary to use an erbium-doped fiber (EDF) as the gain medium. Moreover, to eliminate mode conversion loss in the fiber and avoid the required multi-mode pump, it is better to use a single-mode EDF (SM-EDF), rather than a multi-mode EDF.
In this paper, based on the SM-EDF as well as an SLM, a fiber laser for prescribing pure higher-order laser modes in the 1550 nm band is proposed. The SLM is designated to generate higher-order modes. By setting the SLM, the desired higher-order mode and the fundamental mode presenting a different diffraction angle can be separated. Then, the wanted higher-order mode can be exported, while the residual fundamental mode is coupled into the fiber ring oscillator again. As we all know, the fundamental mode, and not the higher-order mode, is a self-reproducing mode of the fiber ring laser. By virtue of this simple and critical design, the gain medium (i.e., the EDF) can be pumped by the single-mode 980 nm laser, no matter what higher-order mode is prescribed. Furthermore, the proposed method is important because the requirement for a higher-order mode pump is avoided, which is usually necessary for mode amplification in SDM optical fiber communications. Simultaneously, the mode conversion loss is also greatly reduced because the fundamental mode oscillates in the fiber laser. Notice that the concept of the superpixel is used in the SLM to enhance the system performance. In conclusion, high-quality higher-order modes from to are observed in our proof-of-principle experiment.
2. REALIZATION OF THE ON-DEMAND MODE LASER
The experimental setup is shown in Fig. 1. The laser cavity is a ring cavity with SM-EDF acting as the gain medium. The pump light (980 nm) and oscillating light (1550 nm) are coupled into the SM-EDF through a wavelength-division multiplexing (WDM) coupler. The length of the SM-EDF is 10 m. In the SM-EDF, most of the pump light is absorbed and the oscillating light is amplified. After the amplification in the SM-EDF, the light field enters into free space for wavelength filtering and mode control. The center wavelength of the wavelength filter is . The half-wave bandwidth of the wavelength filter is . Therefore, the wavelength is locked in the C and L bands. Then, after the beam passes the half-wave plate (HWP) and polarizer, the polarization direction of the beam is consistent with the functional direction of the SLM, and thus the beam will be shaped by the SLM. The role of the SLM is to load a multi-mode mask for the excitation of on-demand higher-order modes. After the modulation of the multi-mode mask, the beam is split into two sub-beams (one beam with the mode pattern and one beam with a higher-order mode pattern). The mode beam is then coupled into a single-mode fiber and amplified again in the SM-EDF. The higher-order mode beam, however, is coupled into a few-mode fiber (FMF) for transmission. The isolators guarantee the light field in the cavity for one-way transmission only.
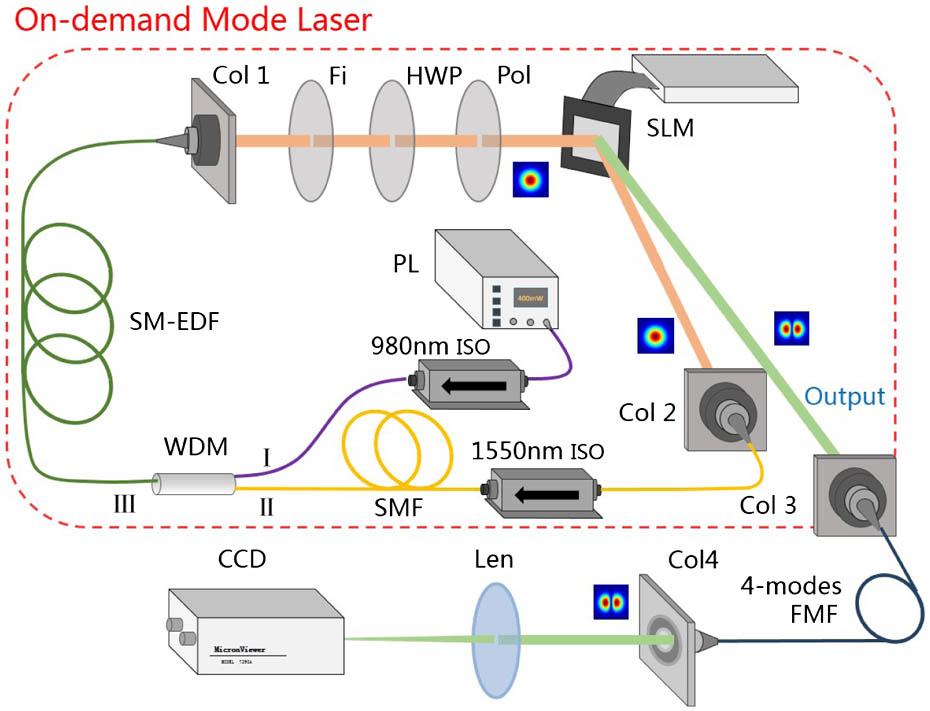
Figure 1.Experimental setup of on-demand mode laser. PL, 980 nm pump laser; ISO, isolator; Col, fiber collimator; Fi, filter; Pol, polarizer; SMF, single-mode fiber.
A multi-mode mask capable of simultaneously exciting several modes is utilized in our scheme. The expression of the multi-mode mask is where () denotes the phase of the desired modes and () denotes a blazed grating being added to each desired mode. The expression of the blazed grating iswhere denotes the position along the horizontal or vertical axis, denotes the pixel pitch of the SLM, denotes the grating period, and mod denotes the modulus function. Different blazed gratings make each desired mode present a different propagation direction.
In the experimental scheme, and are set. Thus, Eq. (1) is simplified as follows:
After using the multi-mode mask, part of the light is still in the mode without being shaped. However, other light is shaped and then converted into a higher-order mode. The propagation direction of the higher-order mode is changed, while that of is not. The coefficients and in Eq. (3) are related to the power distribution between modes. Parameters of and are set to achieve a power balance between two modes.
In Eq. (1), is defined as the phase of the desired modes. However, the converted mode after pure phase modulation is not exactly the same as the desired mode. The difference lies in the amplitude distribution. Thus, the method of the superpixel that realizes combined amplitude and phase modulation is utilized to generate . Superpixels correspond to small areas on the SLM, each of which has a checkerboard pattern with two independent pixel values [Fig. 2(a)]. After being reflected by the superpixel, the intensity of the light can be controlled by the difference in the pixel values, and the phase shift can be set through the individual phase shifts. In previous work, the intensity modulation function of our SLM was measured [16], and the expression is where [Fig. 2(a)] denotes the difference between two independent pixel values. The multi-mode mask for the excitation of the mode using the superpixel is shown in Fig. 2(b). The simulation results clearly demonstrate the feasibility of our scheme for the simultaneous and accurate excitation of the and modes [Fig. 2(c)]. At the same time, the excitation of the and modes is also given [Figs. 2(d) and 2(e)].
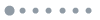
Figure 2.(a) Phase setting of superpixel cell, (b) multi-mode mask optimized by superpixel for excitation of the mode, (c) the simulation results of the simultaneous excitation of the and modes, (d), (e) the simulation results for the generation of the and modes, respectively, (f) the simultaneous excitation of the and modes by using the multi-mode mask in the experiment.
In addition, the excitation of higher-order modes is demonstrated in the experiment. The experimental results of simultaneous excitation of the and modes are shown in Fig. 2(f). By controlling the multi-mode masks loaded on the SLM in real time, the excitation of other higher-order modes is also demonstrated (Fig. 3). The output mode fields from the laser are coupled into four-mode FMF in transmission. The intensity distribution of the output beam after propagating 100 m in FMF is captured using CCD. The intensity distributions of the modes before and after propagation in FMF are shown in Fig. 4.
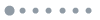
Figure 3.Examples of higher-order mode output from the on-demand mode laser.
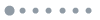
Figure 4.Optical intensity distributions of the , , , and modes before and after propagation in four-mode FMF. (a) The intensity distributions before propagation, captured by a CCD located in the focal point of the lens, and (b) the far-field intensity distributions after propagation in 100 m FMF.
In order to analyze the spectrum of the output laser, the multi-mode masks for the excitation of two modes are loaded onto the SLM. Then, after injecting light into the optical spectrum analyzer, we obtain the spectrum diagram, as shown in Fig. 5(a). Obviously, the center wavelength obtained and the bandwidth of the output laser are 1575.9 and 1 nm, respectively. In addition, to analyze the polarization state of the output from the fiber laser, the output mode is also coupled into the polarization synthesizer to obtain the polarization data. The output laser is linearly polarized light, and the degree of polarization remains stable (Visualization 1). Finally, for the mode, the quantum efficiency is presented in Fig. 5(b). It can be clearly seen that the output power increases linearly with the pump power. The laser threshold is only 26.6 mW, and the power of the output laser is 52.3 μW. The maximum output power of the mode is 4.196 mW, because the output power is limited by the maximum pump power of 288.2 mW.
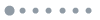
Figure 5.(a) Measured spectrum of the mode generated from the on-demand mode laser and (b) the quantum efficiency of the mode.
3. DISCUSSION
The SLM used in the experiment contains 1920×1080 pixels with an 8 μm pixel pitch and 256 (8 bit) gray level. The resolution is sufficient to achieve the accurate excitation of many lower-order modes. The experimental results are clear, with a weak distortion at the periphery of the excited modes (Figs. 3 and 4). The weak distortion can be filtered out through beam propagation in FMF (Fig. 4) or by using a filter system. For higher-order modes ( and , for example), a bright spot exists at the center of the light field, which does not appear in the simulation results shown in Figs. 2(d) and 2(e). It can be derived from the inaccurate modulation of the SLM and can be eliminated by utilizing a SLM with high modulation accuracy or phase plates.
In a few-mode erbium-doped fiber amplifier (FM-EDFA), to boost the power of signals carried by modes, pumping in a higher-order mode is preferred to significantly reduce the mode-dependent gain. Before coupling into the FM-EDFA, the 980 nm pump light is transformed by an optional phase plate [17]. To get rid of the requirement on the higher-order mode pump and mode conversion, the SM-EDF is the best choice, and also greatly reduces the loss in the laser cavity. Under sufficient pump power and the proper doping concentration of erbium ions in SM-EDF, the gain of a 1550 nm laser can well overcome the loss of the ring cavity. Finally, the stable output of the higher-order modes is achieved.
4. CONCLUSION
In conclusion, we propose a method to effectively excite transverse laser modes based on an SM-EDF and an SLM. The SM-EDF is employed to be the gain medium, and the SLM is designated to convert the fundamental mode to the desired higher-order mode. The desired higher-order mode is exported, while the residual fundamental mode is coupled into the ring cavity. In general, the experimental implementation is simple but efficient. The propagation of the , , , and modes from a laser in a four-mode FMF is also demonstrated. Because of it characteristics of being on-demand and flexible, the on-demand laser will play an important role in a variety of interesting applications.
Acknowledgment
Acknowledgment. The authors thank the FiberHome Corporation for providing single-mode erbium-doped fiber and four-mode few-mode fiber in the experiment. We also thank Liaocheng University for providing the spatial light modulator in the test.
References
[1] G. Li, N. Bai, N. Zhao, C. Xia. Space-division multiplexing: the next frontier in optical communication. Adv. Opt. Photon., 6, 413-487(2014).
[2] I. Spaleniak, S. Gross, N. Jovanovic, R. J. Williams, J. S. Lawrence, M. J. Ireland, M. J. Withford. Multiband processing of multimode light: combining 3D photonic lanterns with waveguide Bragg gratings. Laser Photon. Rev., 8, L1-L5(2014).
[3] H. Huang, G. Milione, M. P. J. Lavery, G. Xie, Y. Ren, Y. Cao, N. Ahmed, T. A. Nguyen, D. A. Nolan, M. Li, M. Tur, R. R. Alfano, A. E. Willner. Mode division multiplexing using an orbital angular momentum mode sorter and MIMO-DSP over a graded-index few-mode optical fibre. Sci. Rep., 5, 14931(2015).
[4] A. Li, Y. Wang, Q. Hu, W. Shieh. Few-mode fiber based optical sensors. Opt. Express, 23, 1139-1150(2015).
[5] Y. Weng, E. Ip, Z. Pan, T. Wang. Single-end simultaneous temperature and strain sensing techniques based on Brillouin optical time domain reflectometry in few-mode fibers. Opt. Express, 23, 9024-9039(2015).
[6] A. Forbes, A. Dudley, M. McLaren. Creation and detection of optical modes with spatial light modulators. Adv. Opt. Photon., 8, 200-227(2016).
[7] L. Cui, J. Su, X. Li. Distribution of entangled photon pairs over few mode fibers. Asia Communications and Photonics Conference, AF1D.5(2016).
[8] J. Hamazaki, R. Morita, K. Chujo, Y. Kobayashi, S. Tanda, T. Omatsu. Optical-vortex laser ablation. Opt. Express, 18, 2144-2151(2010).
[9] D. J. Richardson, J. M. Fini, L. E. Nelson. Space-division multiplexing in optical fibres. Nat. Photonics, 7, 354-362(2013).
[10] S. O. Arik, J. M. Kahn. Direct-detection mode-division multiplexing in modal basis using phase retrieval. Opt. Lett., 41, 4265-4268(2016).
[11] S. Gross, N. Riesen, J. D. Love, M. J. Withford. Three dimensional ultra broadband integrated tapered mode multiplexers. Laser Photon. Rev., 8, L81-L85(2014).
[12] A. Li, Y. Wang, J. Fang, M. Li, B. Y. Kim, W. Shieh. Few-mode fiber multi-parameter sensor with distributed temperature and strain discrimination. Opt. Lett., 40, 1488-1491(2015).
[13] J. Leach, M. J. Padgett, S. M. Barnett, S. Franke-Arnold, J. Courtial. Measuring the orbital angular momentum of a single photon. Phys. Rev. Lett., 88, 257901(2002).
[14] W. Koechner. Solid-State Laser Engineering(2006).
[15] S. Ngcobo, I. Litvin, L. Burger, A. Forbes. A digital laser for on-demand laser modes. Nat. Commun., 4, 2289(2013).
[16] C. Ma, S. Yu, M. Lan, S. Cai, S. Nie, W. Gu. Optimization of super pixel for higher order mode excitation in mode-division multiplexing system. Asia Communications and Photonics Conference, ASu2A.97(2015).
[17] N. Bai, E. Ip, Y. Huang, E. Mateo, F. Yaman, M. Li, S. Bickham, S. Ten, J. Liñares, C. Montero, V. Moreno, X. Prieto, V. Tse, K. M. Chung, A. P. T. Lau, H.-Y. Tam, C. Lu, Y. Luo, G.-D. Peng, G. Li, T. Wang. Mode-division multiplexed transmission with inline few-mode fiber amplifier. Opt. Express, 20, 2668-2680(2012).