Abstract
The stimulated Brillouin scattering (SBS) threshold affected by repetition rate and pulse duration in a single-frequency nanosecond pulsed fiber amplifier is studied. The experimental results demonstrate that the SBS threshold can be improved either by reducing the repetition rate or by narrowing the pulse duration; however, the average power may be limited in some cases. Otherwise, two evaluation methods for the SBS threshold in the fiber amplifier are compared and discussed, aiming to obtain a more accurate description for the SBS threshold in our single-frequency amplifier system.High-power nanosecond pulsed laser sources with a single frequency have many important applications, such as material processing, remote sensing, laser radar, nonlinear frequency generation, and coherent beam combination[1–5]. However, for lasers whose bandwidth is narrow compared to the Brillouin linewidth (), also considered as a single frequency, the output amplifier power is clamped when electrostriction creates an acoustic wave in the fiber, leading to backward stimulated Brillouin scattering (SBS) of the signal power[6]. This is a major disruption because it reduces the output optical efficiency and even induces potentially destructive parasitic pulsing behaviors[7]. In addition, the SBS exhibits very high gain (more than two orders of magnitude larger than stimulated Raman scattering), which results in a very low-onset threshold. Therefore, lots of techniques have been proposed to suppress the SBS and increase the output power of a single-frequency fiber amplifier, such as shortening the fiber length and increasing the mode area[8,9], thermal and stress gradients[10,11], low nonlinearity fiber designing[12,13], and multi-tone driving[14,15]. Particularly, in the pulse regime, the SBS threshold also depends on the pulse duration because the response of the fused silica in the SBS case is governed by the acoustic phonon lifetime of , so making the pulse duration less than 10 ns can greatly reduce the SBS gain[16]. This research has attracted much attention in recent years. In 2011, Geng et al.[17] reported a 1.32 kW single-frequency pulsed fiber laser with a pulse width that was kept at to prevent the onset of SBS in a single-mode fiber. However, the temporal characteristics were not researched and the very short active fiber length (20 cm) was also the key factor to suppressing the SBS. Su et al.[18] obtained a 7.9 kW narrow-linewidth pulsed laser with an average power of 505 W when operating in a pulse width, and no SBS was observed. However, the linewidth of the modulated fiber laser seed was a dual-single frequency. To investigate the dynamic process of SBS in a single-frequency pulsed fiber amplifier with a pulse width of less than 10 ns, we set up a series of experiments to study the dependencies of the SBS threshold on the temporal characteristics, including repetition rate and pulse duration. Su et al.[19] studied the impact of temporal characteristics such as pulse width, pulse period and pulse shape on the SBS threshold for a nanosecond laser, but just did a numerical analysis. Before that, they had demonstrated experimental results about the dependencies of the SBS threshold in pulsed fiber amplifiers on spectral linewidth, pulse duration, and repetition rate[20], but the conclusions are not based on lasers with a single frequency.
In this Letter, the SBS threshold in a single-frequency nanosecond pulsed fiber amplifier that depends on the temporal characteristics is demonstrated experimentally. The fiber amplifier system is based on a master oscillator power amplifier (MOPA) structure with an intensity-modulated pulsed seed by an electro-optic modulator (EOM). Pulsed lasers with different pulse durations and repetition rates can be obtained by setting the parameters of a signal generator (SG). The SBS effect can be observed at different power levels under different pulse parameters. It is worth noting that the emergence of SBS can be either evaluated by the backward spectrum or by backward Stokes wave power. These two evaluation methods on the SBS threshold deduce different descriptions of the power handing limitation for the fiber amplifier system, so it is necessary to compare and analyze the two methods in detail.
The experimental setup of the all-fiber single-frequency pulsed fiber amplifier is shown in Fig. 1. The pulse seed consists of a 1064 nm single-frequency CW laser with an output power of and an EOM that has a maximum optical input power of 100 mW and a bandwidth of 10 GHz, which is used to directly modulate the CW fiber laser. An SG outputs an electrical signal with all kinds of pulse durations and repetition rates that is used to drive the fiber-based EOM; then, the pulsed laser seed is obtained. To protect the EOM and the single-frequency CW laser, a 20 W isolator (ISO) is inserted behind the output of the seed. When setting the RF signal generated by the SG with a 10 MHz repetition rate and a 4 ns pulse width, output optical pulses with 0.6 mW average power, a 10 MHz repetition rate, and pulse width are obtained. The linewidths of the CW laser and the pulsed seed laser after modulation are measured by a scanning Fabry–Perot (F–P) interferometer over the free spectral range (FSR) of 4 GHz. The results show that the linewidth is broadened from 24 to 73 MHz, as seen in Fig. 2; thus, , indicating that the single-frequency pulsed laser is transform limited[17]. A pre-amplifier (Amp1) with a 600 mW single-mode laser diode (LD) and a 1 m Yb-doped single-cladding fiber is used to amplify the seed’s average power from 0.6 to 228 mW. The second-stage amplifier (Amp 2) and the third-stage amplifier (Amp3) amplify the pulsed laser to average powers of 5 and 70 W, respectively. The gain fiber of Amp 2 and Amp 3 is a 4 m 10/125 μm Yb-doped fiber (YDF) and a 1.5 m 15/130 μm YDF. Amp 2 is clad pumped by a 9 W multimode fiber pigtailed 976 nm LD via a signal/pump combiner, and Amp 3 is clad pumped by three 27 W LDs via a combiner. ISOs are spliced in each stage to protect the components from backward lights such as an amplified spontaneous emission (ASE). A 10 W tap is inserted behind ISO2 to detect the backward light of Amp3. If the SBS threshold is achieved, the output power monitored from port P1 of the tap will increase nonlinearly and the backward Stokes peak will also be observed by a spectroscope connected to port P1. An angle is polished to avoid a Fresnel reflection.
Sign up for Chinese Optics Letters TOC. Get the latest issue of Chinese Optics Letters delivered right to you!Sign up now
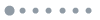
Figure 1.Experimental arrangement of the all-fiber single-frequency pulsed fiber amplifier.
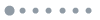
Figure 2.Spectra of the (a) CW laser and (b) intensity-modulated pulsed laser over an F–P interferometer.
Since the first-order Stokes line centered at 0.06 nm red-shifted from the pump wave for the pump wavelength of 1064.4 nm is observed while the pulsed average laser power is being amplified to 70 W, the SBS threshold for a nanosecond laser being amplified in a 15/130 μm fiber should be investigated in detail. The two evaluation methods for the SBS threshold are defined as follows: (1) as soon as the Stokes line in the backward spectrum is observed, the corresponding amplified peak power is considered to be the SBS threshold power; and (2) as the backward Stokes average power increases to 2% of the forward average pump power, the peak pump power is thought to be the SBS threshold power. Here, we just take the Amp3 stage into consideration for the SBS effect, because the power of formal stages is lower than the SBS threshold. Since the total fiber length of Amp3 is 2.5 m, the backward Stokes wave generated by the pump pulse at the exit end of the fiber will get out from the other port after 12 ns (, is the refractive index in the fiber). So, in order to prevent the Stokes pulse not only from interacting with the pump pulse generated by itself, but also with the other pump pulses that arrive later, the repetition rate needs to be less than 40 MHz in our system. First, we maintain the pulse duration of the driven signal to be 4 ns and set the repetition rates to be 5, 10, and 15 MHz. The SBS thresholds under these situations are compared in both evaluation methods, as shown in Figs. 3 and 4. It can be seen that the first-order Stokes line appears when the average power is amplified to 36 W and rises quickly, with the pump power increasing for the repetition rate of 5 MHz. The average power point is 50 W when the repetition rate is 10 MHz. At a repetition rate of 15 MHz, no apparent Stokes line is observed, even with the average power being amplified to 70 W. However, the SBS thresholds in pulsed fiber lasers always refer to the peak power, which is evaluated after considering the CW component in the fiber. The corresponding SBS threshold according to the spectra in Figs. 3(a)–3(c) is 1160, 815, and , respectively. So by using the first evaluation method, the SBS threshold can be improved by reducing the repetition rate, but the average output power is limited. Adopting the backward Stokes wave power to evaluate the SBS threshold as shown in Fig. 4, the average power points for SBS initiating are 30.2, 36.3, and 36.3 W corresponding to repetition rates of 5, 10, and 15 MHz. So the corresponding SBS thresholds are 924, 603, and 413 W with the repetition rate increasing. Every data point in Fig. 4 is measured three times to make sure it is accurate and that the mean value is adopted. By comparing the two evaluation methods, we can see that the changing trend of the SBS threshold depends upon a repetition rate that is almost the same, but the average output power exhibits different limitations. The average power points are basically same as each other regardless of whether the repetition rate is higher or lower when considering the backward Stokes power. Thus, the power handing limitation refers to the peak power.
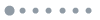
Figure 3.Backward spectra under different average output powers with repetition rates of (a) 5, (b) 10, and (c) 15 MHz.
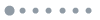
Figure 4.Backward Stokes average power versus average output pump power with repetition rates of 5, 10, and 15 MHz.
To analyze the effects of pulse duration on the SBS threshold, the repetition rate of the signal pulse is maintained at 10 MHz and the pulse duration is set to 4, 8, and 10 ns. The pulse widths of the obtained pulsed laser are 5.5, 9.2, and 11.1 ns. The SBS thresholds evaluated by the above two methods are depicted in Figs. 5 and 6. The average power points for the generation of the SBS in Figs. 5(a) and 5(b) are both 50 W when the pulse width is less than 10 ns. But when the pulse width is larger than 10 ns, as shown in Fig. 5(c), the average power point is decreased to 44 W, and the intensity of Stokes line increases more quickly than in the 4 and 8 ns situations. The corresponding SBS thresholds of 4, 8, and 10 ns are 815, 485, and 363 W, respectively. This implies that the SBS threshold increases by decreasing the pulse width of the signal laser. By adopting the second evaluation method, the SBS threshold decreases from 603 to 290 and 209 W when the pulse duration of the electrical signal is increased from 4 to 8 and 10 ns, as shown in Fig. 6. The average power points for the corresponding SBS threshold are 36.3, 30.3, and 30.3 W. So, the two evaluation methods both prove the deduction that a narrower pulse width results in a higher SBS threshold. The average power points for the SBS threshold also show the same limitation trends, that a higher average power will be obtained with a narrower pulse width.
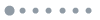
Figure 5.Backward spectra under different average output powers with pulse durations of (a) 4, (b) 8, and (c) 10 ns.
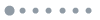
Figure 6.Backward Stokes average power versus average output pump power with repetition rates of 4, 8, and 10 ns.
Among all the above situations, the output pulse shapes and pulse widths during the amplifying processes remain stable and the signal-to-noise ratio of the forward output optical spectra are controlled to be higher than 40 dB. Considering the average power and peak power comprehensively, a repetition rate of 10 MHz and a pulse duration of 4 ns is the best choice. When the average output power is amplified to 70 W, the obtained peak power is 1.15 kW and the pulse width of the signal laser is 5.5 ns. The spectral linewidth of the amplified laser is measured to be , which is broadened by a factor of 5.6. We attribute the spectral broadening to the nonlinear effect of self-phase modulation (SPM) in the fiber[17], since the laser pulse width is not reduced during the whole pulse amplification and the estimated value of the maximum nonlinear phase shift induced by the SPM is calculated to by the formula of , where is the nonlinear index coefficient, is the fiber length, is the laser intensity, and is the laser peak power. is the mode field radius and is the laser’s central wavelength. The pulse shape and the forward optical spectrum are shown in Figs. 7 and 8.
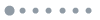
Figure 7.Typical pulse shapes of the amplified pulses with an average power of 70 W.
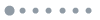
Figure 8.Forward optical spectrum of the amplified pulsed laser with an average power of 70 W.
In conclusion, we demonstrate a single-frequency nanosecond MOPA laser in an all-fiberized structure with kilowatt peak power. The SBS thresholds evaluated by two methods are discussed in detail when setting the pulsed laser at different repetition rates and pulse durations. The thresholds are highly related to the temporal characteristics regardless of whether we are considering the backward spectra or the backward Stokes wave power. Pulses with lower repetition rates and narrower pulse durations result in lower SBS thresholds. However, the average output power will be limited if the repetition rate is too low in a certain fiber system because the Stokes line will appear at a lower average power point and increase quickly in the backward spectrum compared with higher repetition rate pulses. So, in order to obtain a higher average power and peak power together when the pulse duration is less than 10 ns, we need to balance the increment of the peak power against the decrement of the average power for lower repetition rates before the Stokes line rises.
References
[1] F. Di Teodoro, P. Belden, P. Ionov, N. Werner. Opt. Fiber Technol., 20, 688(2014).
[2] V. Philippov, C. Codemard, Y. Jeong, C. Alegria, J. K. Sahu, J. Nilsson. Opt. Lett., 29, 2590(2004).
[3] W. Diao, X. Zhang, J. Liu, X. Zhu, Y. Liu, D. Bi, W. Chen. Chin. Opt. Lett., 12, 072801(2014).
[4] C. E. Dilley, M. A. Stephen, M. P. Savage-Leuchs. Opt. Express, 15, 14389(2007).
[5] H. Liu, B. He, J. Zhou, J. Dong, Y. Wei, Q. Lou. Opt. Lett., 37, 3885(2012).
[6] J. W. Dawson, M. J. Messerly, R. J. Beach, M. Y. Shverdin, E. A. Stappaerts, A. K. Sridharan, P. H. Pax, J. E. Heebner, C. W. Siders, C. P. J. Barty. Opt. Express, 16, 13240(2008).
[7] H. Injeyan, G. Goodno. High Power Laser Handbook(2011).
[8] W. Shi, E. B. Petersen, M. Leigh, J. Zong, Z. Yao, A. Chavez-Pirson, N. Peyghambarian. Opt. Express, 17, 8237(2009).
[9] Q. Fang, W. Shi, K. Kieu, E. Petersen, A. Chavez-Pirson, N. Peyghambarian. Opt. Express, 20, 16410(2012).
[10] J. Hansryd, F. Dross, M. Westlund, P. A. Andrekson, S. N. Knudsen. J. Lightwave Technol., 19, 1691(2001).
[11] L. Zhang, J. Hu, J. Wang, Y. Feng. Opt. Lett., 37, 4796(2012).
[12] J. Liu, J. Zhang, J. Han, G. Gao, Y. Zhao, W. Gu. Chin. Opt. Lett., 12, 030601(2014).
[13] S. Du, Z. Wang, Z. Wang, J. He, J. Zhou, Q. Lou. Chin. Opt. Lett., 11, 091402(2013).
[14] I. Dajani, C. Zeringue, T. M. Shay. IEEE J. Sel. Top. Quantum Electron., 15, 406(2009).
[15] P. Weßels, P. Adel, M. Auerbach, D. Wandt, C. Fallnich. Opt. Express, 12, 4443(2004).
[16] G. P. Agrawal. Nonlinear Fiber Optics(2006).
[17] J. Geng, Q. Wang, Z. Jiang, T. Luo, S. Jiang, G. Czarnecki. Opt. Lett., 36, 2293(2011).
[18] R. T. Su, X. L. Wang, P. Zhou, X. J. Xu. Laser Phys. Lett., 10, 015105(2013).
[19] R. Su, P. Zhou, H. Lü, X. Wang, C. Luo, X. Xu. Opt. Commun., 316, 86(2014).
[20] R. Su, P. Zhou, X. Wang, H. Xiao, X. Xu. Chin. Opt. Lett., 10, 111402(2012).