
- Chinese Optics Letters
- Vol. 20, Issue 3, 031408 (2022)
Abstract
1. Introduction
High-power lasers are widely used in industrial and scientific fields. In recent years, solid-state lasers[
The main factor limiting the improvement of laser power is the volume of gain medium. The previous theoretical model suggested that a discharge volume of tens of cubic centimeters would be required for a 100 kW laser system[
2. Experimental Setup
A discharge chamber (with inner diameter 200 mm and height 400 mm) of stainless steel six-way cross was constructed with four windows on the sides, which were anti-reflection coated for the IR radiation. A turbo pump (SL300, Leybold) at the top of the vacuum chamber was used to evacuate the chamber to
Sign up for Chinese Optics Letters TOC. Get the latest issue of Chinese Optics Letters delivered right to you!Sign up now
Figure 1.Schematic of the plasma generator and the setup for laser diode absorption spectroscopy. LD, laser diode; SP, monochromator; PMT, photomultiplier tube; CCPS, capacitor charging power supply; MOSFET, metal-oxide-semiconductor field-effect transistor.
A home-made narrow linewidth (10 GHz) diode laser[
3. Experimental Results and Discussion
The temporal waveforms of the voltage and current measured at point A marked in Fig. 1 are shown in Fig. 2. In the experiments, high-voltage pulses were adjusted by the voltage setting of a capacitor charging power supply (CCPS), and the maximum value of the pulsed voltage was slightly less than two times the charged voltage at the storage capacitors C1 and C2 with capacitance 4 nF each. The related electric energy deposited into the plasma, calculated by integrating the product of the current and voltage measured at point A, was about 2.1 mJ. The current generated by the discharge device is one order of magnitude higher than that of Mikheyev et al.[
Figure 2.Typical traces of pulsed voltage and current with gas mixture of 1% Ar/He at 800 mbar, of which the deposited energy is about 2.1 mJ. CCPS is set at 800 V, with the voltage at C1 and C2 charged to 1.33 kV.
Photos of discharge plasma along the
Figure 3.Plasma photos taken along the (a) y direction and (b) x direction at 800 mbar. Two segmented electrodes were used each with a length of 25 mm in the x direction and a width of 8 mm in the y direction.
In the experiments, we investigated the dependence of the density of metastable particles on the charging voltage by changing the voltage set at the CCPS, as shown in Fig. 4(a). The probing beam entered the plasma in a segment along the
Figure 4.(a) Curves of Ar (1s5) density versus time at different DC charging voltages and 800 mbar. An absorption length of 8 mm was used. (b) Voltage at the output of pulsed DC power supply at different DC charging voltages and 800 mbar.
High pressure is necessary for OPRGLs to improve the rates of relaxation between energy levels and broaden the absorption linewidth of metastable atoms for the pumping. However, it is noted that the breakdown voltage increases with the gas pressure, which affects the generation of metastable atoms. We investigated the effect of gas pressure on the generation of metastable atoms. As shown in Fig. 5, the peak value of the density of metastable atoms does not change significantly with the increase of pressure, while the lifetime of metastable atoms decreases significantly with the increase of pressure. Therefore, high repetition rate [perhaps higher than 100 kHz, according to the duration of the metastable states shown in Fig. 4(a)] is required to continuously produce high-density metastable states in the segmented pulsed discharge at atmospheric pressure.
Figure 5.Dependence of Ar (1s5) density on pressure. CCPS is set at 800 V. An absorption length of 8 mm was used.
We investigated the temporal evolution of the characteristic emission lines of the gas mixture. The wavelength and related energy levels of the emission lines are shown in Table 1. Figure 6 shows that the temporal waveforms of emission lines exhibit a bimodal shape, indicating that the dominant mechanism leading to the generation of metastable atoms in such a pulsed discharge with high deposited energy is divided into two periods in time. Note that the pulsed current in Fig. 2 almost vanished in 1 µs after the gas mixture got broken down. It can be seen from the temporal waveforms of different emission lines that a large number of active particles such as metastable Ar atoms, excited Ar atoms, and Ar ions were generated in the first duration of 100 ns. After the pulsed current almost vanished, there was still afterglow, which indicated that when the electron excitation turned weak, the decay and relaxation collisions took the major role in the generation of metastable particles. In addition, since the intensity of emission is proportional to the occupation density of the upper level and the spontaneous emission coefficient, we noticed that the particle densities in the highly excited states of Ar (such as 2p1 and 2p2), which were mainly produced by ion recombination and relaxation collision, were quite considerable compared to that of 2p9. Therefore, it is necessary to modify the pulsed discharge models[
Figure 6.Time-resolved intensity of characteristic emission of Ar atoms at 800 mbar. The DC charging voltage is 750 V.
Wavelength (nm) | Type | Transition Levels | Spontaneous Emission Coefficient ( |
---|---|---|---|
418.19 | Ar I | ||
419.83 | Ar I | ||
420.07 | Ar I | ||
425.94 | Ar I | ||
433.36 | Ar I | ||
696.54 | Ar I | ||
750.39 | Ar I | ||
801.48 | Ar I | ||
811.53 | Ar I | ||
826.45 | Ar I | ||
447.48 | Ar II | ||
473.59 | Ar II | ||
501.72 | Ar II |
Table 1. Wavelength and Transition Levels of Each Selected Line[27]
4. Conclusions
The primary objective of this study was to investigate the possibility of whether a segmented pulsed discharge could be used to realize a volume-scalable structure with high densities of Ar (1s5) metastable particles. This segmented discharge structure with peaking capacitors eliminated the need of separated drive circuits, reducing the complexity and cost of design. The peaking capacitors were charged by a DC pulsed power supply, and a narrow and intense pulsed current was generated every time when the discharge was triggered. Calculated from the measured voltage and current waveforms at the outlet of the pulsed power supply, the highest energy deposited into the discharge zone of
In fact, the practicability of this structure is far more than a one-dimensional arrangement. In subsequent experiments, the possibility of the two-dimensional array structure for further expanding the volume of dense plasma will be tested.
References
[1] M. Zhao, C. Wang, Q. Hao, Z. Zou, J. Liu, X. Fan, L. Su. High single-pulse energy passively Q-switched laser based on Yb,Gd:SrF2 crystal. Chin. Opt. Lett., 18, 101401(2020).
[2] R. Lü, H. Teng, J. Zhu, Y. Yu, W. Liu, G. Chang, Z. Wei. High power Yb-fiber laser amplifier based on nonlinear chirped-pulse amplification at a repetition rate of 1 MHz. Chin. Opt. Lett., 19, 091401(2021).
[3] H. Chen, X. Jiang, S. Xu, H. Zhang. Recent progress in multi-wavelength fiber lasers: principles, status, and challenges. Chin. Opt. Lett., 18, 041405(2020).
[4] J. W. Dawson, M. J. Messerly, R. J. Beach, M. Y. Shverdin, E. A. Stappaerts, A. K. Sridharan, P. H. Pax, J. E. Heebner, C. W. Siders, C. P. J. Barty. Analysis of the scalability of diffraction-limited fiber lasers and amplifiers to high average power. Opt. Express, 16, 13240(2008).
[5] B. V. Zhdanov, R. J. Knize. Review of alkali laser research and development. Opt. Eng., 52, 021010(2013).
[6] R. J. Beach, W. E. Krupke, V. K. Kanz, S. A. Payne, M. A. Dubinskii, L. D. Merkle. End-pumped continuous-wave alkali vapor lasers: experiment, model, and power scaling. J. Opt. Soc. Am. B, 21, 2151(2004).
[7] J. Han, M. C. Heaven. Gain and lasing of optically pumped metastable rare gas atoms. Opt. Lett., 37, 2157(2012).
[8] A. V. Demyanov, I. V. Kochetov, P. A. Mikheyev. Kinetic study of a cw optically pumped laser with metastable rare gas atoms produced in an electric discharge. J. Phys. D, 46, 375202(2013).
[9] J. Han, M. C. Heaven, P. J. Moran, G. A. Pitz, E. M. Guild, C. R. Sanderson, B. Hokr. Demonstration of a CW diode-pumped Ar metastable laser operating at 4 W. Opt. Lett., 42, 4627(2017).
[10] P. J. Moran, N. P. Lockwood, M. A. Lange, D. A. Hostutler, E. M. Guild, M. R. Guy, J. E. McCord, G. A. Pitz. Plasma and laser kinetics and field emission from carbon nanotube fibers for an advanced noble gas laser (ANGL). Proc. SPIE, 9729, 97290C(2016).
[11] W. T. Rawlins, K. L. Galbally-Kinney, S. J. Davis, A. R. Hoskinson, J. A. Hopwood, M. C. Heaven. Optically pumped microplasma rare gas laser. Opt. Express, 23, 4804(2015).
[12] Z. Yang, G. Yu, H. Wang, Q. Lu, X. Xu. Modeling of diode pumped metastable rare gas lasers. Opt. Express, 23, 13823(2015).
[13] B. Eshel, G. P. Perram. Five-level argon-helium discharge model for characterization of a diode-pumped rare-gas laser. J. Opt. Soc. Am. B, 35, 164(2018).
[14] C. R. Sanderson, C. W. Ballmann, J. Han, A. B. Clark, B. H. Hokr, K. G. Xu, M. C. Heaven. Demonstration of a quasi-CW diode-pumped metastable xenon laser. Opt. Express, 27, 36011(2019).
[15] P. A. Mikheyev, A. K. Chernyshov, M. I. Svistun, N. I. Ufimtsev, O. S. Kartamysheva, M. C. Heaven, V. N. Azyazov. Transversely optically pumped Ar:He laser with a pulsed-periodic discharge. Opt. Express, 27, 38759(2019).
[16] H. Kim, J. Hopwood. Scalable microplasma array for argon metastable lasing medium. J. Appl. Phys., 126, 163301(2019).
[17] P. A. Mikheyev, J. Han, A. Clark, C. Sanderson, M. C. Heaven. Production of Ar and Xe metastables in rare gas mixtures in a dielectric barrier discharge. J. Phys. D, 50, 485203(2017).
[18] A. R. Hoskinson, J. Gregório, J. Hopwood, K. L. Galbally-Kinney, S. J. Davis, W. T. Rawlins. Spatially resolved modeling and measurements of metastable argon atoms in argon-helium microplasmas. J. Appl. Phys., 121, 153302(2017).
[19] J. Han, L. Glebov, G. Venus, M. C. Heaven. Demonstration of a diode-pumped metastable Ar laser. Opt. Lett., 38, 5458(2013).
[20] Z. Zhang, P. Lei, Z. Song, P. Sun, D. Zuo, X. Wang. Optically pumped argon metastable laser with repetitively pulsed discharge in a closed chamber. J. Appl. Phys., 129, 143103(2021).
[21] A. Fridman, A. Chirokov, A. Gutsol. Non-thermal atmospheric pressure discharges. J. Phys. D, 38, R1(2005).
[22] R. Chang, S. Shaw. Compact high-efficiency CO2 slab waveguide lasers with segmented RF excitation. Jpn. J. Appl. Phys., 40, 4552(2001).
[23] J. D. Strohschein, W. D. Bilida, H. J. J. Seguin, C. E. Capjack. Enhancing discharge uniformity in a multi-kilowatt radio frequency excited CO2 slab laser array. Appl. Phys. Lett., 68, 1043(1996).
[24] E. A. Shershunova, V. Y. Khomich, M. V. Malashin, S. I. Moshkunov. Time-space radial development of nanosecond dielectric barrier discharge in flat air gaps under atmospheric pressure. J. Phys. Conf. Ser., 982, 12014(2018).
[25] F. E. Sanz, J. M. G. Perez. Peaking capacitor in an incomplete corona surface discharge preionized TEA CO2 laser. IEEE J. Quantum Electron., 27, 891(1991).
[26] J. Gao, D. Zuo, J. Zhao, B. Li, A. Yu, X. Wang. Stable 811.53 nm diode laser pump source for optically pumped metastable Ar laser. Opt. Laser Technol., 84, 48(2016).
[27] A. Kramida, Y. Ralchenko, J. Reader, N. A. Team. NIST Atomic Spectra Database (ver. 5.8)(2020).
[28] P. Sun, D. Zuo, P. A. Mikheyev, J. Han, M. C. Heaven. Time-dependent simulations of a CW pumped, pulsed DC discharge Ar metastable laser system. Opt. Express, 27, 22289(2019).
[29] D. J. Emmons, D. E. Weeks. Kinetics of high pressure argon-helium pulsed gas discharge. J. Appl. Phys., 121, 203301(2017).
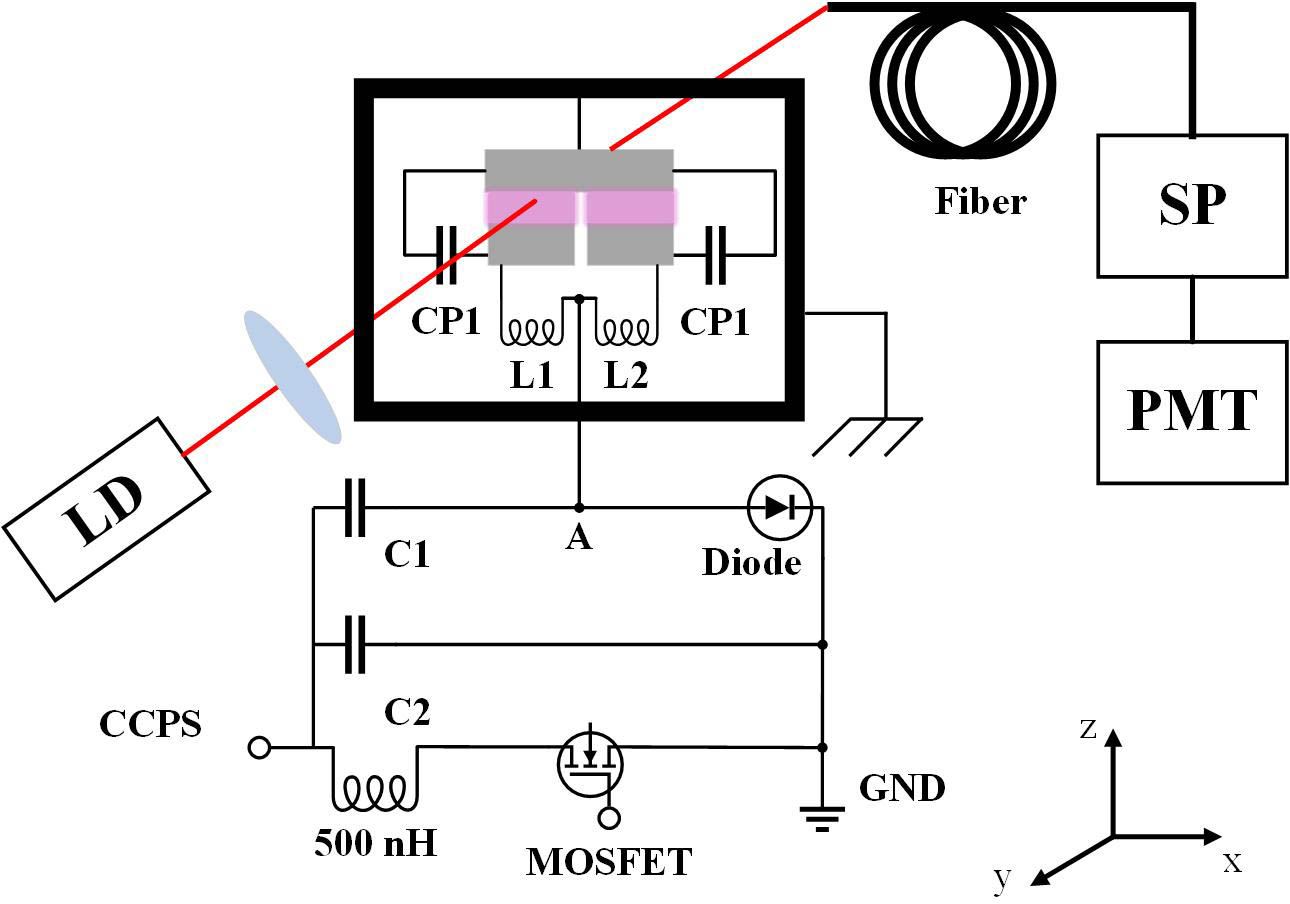
Set citation alerts for the article
Please enter your email address