Abstract
A unique all-fiber interferometric sensor was proposed and successfully demonstrated efficient low-refractive-index liquid sensing in the range from 1.33 to 1.37, which is compatible with those of bio-liquids. A special silica coreless optical fiber with an open V-groove was used as an optical sensing medium, which provided a high sensitivity for a minute liquid volume in the nanoliter scale. The V-groove fiber (VGF) was serially concatenated between two single-mode fibers (SMFs). The LP01 mode guided along the input SMF excited the higher-order modes in the VGF to generate multimode interference, whose spectrum was transmitted through the output SMF. A single liquid droplet with volume of nanoliters wet the entire hydrophilic surface of the VGF, and the transmission spectra shifted corresponding to its refractive index in a very linear manner. The sensor also showed a negligible temperature cross-sensitivity in the range 25°C–75°C, which overlaps with the biological temperature window such that the sensitivity of 159.696 nm per refractive index unit (nm/RIU) remained independent of the temperature variation. Modal properties of VGF were thoroughly analyzed numerically, and detailed processes for the sensor fabrication and sensing experiments were reported.
1. INTRODUCTION
Among various types of optical fiber sensors, refractive-index (RI) sensors have received the most recent attention due to their high potential in emerging biochemical and medical applications [1–4]. In addition to their intrinsic advantages of compact size, remote sensing capability, immunity to electromagnetic interference, and low cost, fiber-optic RI sensors have demonstrated a high sensitivity and a fast response, outperforming electronic alternatives. Since Kumar et al. [5] described the first all-fiber refractometer based on a tapered multimode fiber, various technologies have been adopted in fiber-optic RI sensors such as fiber Bragg grating (FBG) [6–8], tilted fiber Bragg grating (TFBG) [9–11], long period grating (LPG) [12–14], tapered fibers [15,16], Fabry–Perot interferometers [17–19], Mach–Zehnder interferometers [20,21], multimodal interferometers [22–24], surface plasmon resonance (SPR) [25–29], and localized surface plasmon resonance (LSPR) [30–33], to name a few.
LPGs and TFBGs utilize coupling to the cladding modes, which are intrinsically sensitive to the surrounding RI. Meanwhile, FBGs are based on core mode coupling such that FBG RI sensors have required precise removal of the cladding by either etching or polishing to expose the core to the measurands [5,6]. These grating technologies have suffered from cross-sensitivity between the temperature and RI, which limited their applications to only constant-temperature environments [7,8]. Tapered fibers can be formed easily using commercially available equipment; however, it is difficult to precisely control the shape of the taper and maintain a high repeatability in the fabrication process [15,16]. SPR and LSPR technologies, on the other hand, required a thin metal coating on an optically flat surface, and the metal layer’s uniformity and mechanical stability can seriously affect the sensor performances [25–33]. Fiber-optic Fabry–Perot interferometers also have required non-trivial fabrication processes to ensure a high value in a cavity [17–19]. Multimodal interference (MMI) along the concatenated all-fiber structures such as single-mode fiber (SMF)–multimode fiber (MMF)–SMF and SMF-coreless silica fiber (CSF)–SMF, has shown a high potential to overcome prior shortcomings by securing both a high RI sensitivity and negligible temperature–RI cross-sensitivity [22–24]. However, prior SMF–MMF–SMF and SMF–CSF–SMF MMI sensors have been immersed in a designated separate liquid bath containing the liquid volume over a few tens of milliliters at least. This liquid bath inevitably represents only the average RI of the liquid specimen, which cannot cope with the growing demands in the emerging biochemical and medical applications requiring real-time, in situ, single-drop RI sensing capability.
Here, we report a new all-fiber MMI RI sensor by employing a silica V-groove fiber (VGF) as the sensing medium. VGF has been successfully employed to guide the light along the liquid core dispensed along the V groove, providing an intrinsically high birefringence due to the asymmetric structure of VGF [34]. We apply the unique structure of VGF to accurately measure the RI of biocompatible liquid whose volume is in the nanoliter scale for the first time to the best knowledge of the authors.
Sign up for Photonics Research TOC. Get the latest issue of Photonics Research delivered right to you!Sign up now
The proposed fiber-optic RI sensor was fabricated by serially concatenating the input SMF–VGF–output SMF as schematically illustrated in Fig. 1(a). Silica VGF had an open V-shaped groove, and a cross section of a fabricated VGF is shown in Fig. 1(b). In prior studies [20–24], it is well known that the length of an interfering segment in a fiber optic interferometer will determine the spectral range and the interference visibility therein. In our study, we adjusted the CSF length experimentally to be 4.6 cm to obtain the optimal visibility of the output interference in the spectral range covering the C band. In the groove of VGF, a liquid volume of nanoliters can be contained. Note that the inner silica surface of the V-groove had an inherently high hydrophilic nature such that either aqueous or alcoholic liquid can be contained without any airgaps. The liquid within the V-groove interacts with the guided light, and the refractive index of the liquid shifts the MMI spectra, enabling highly sensitive RI sensing in the nanoliter scale.
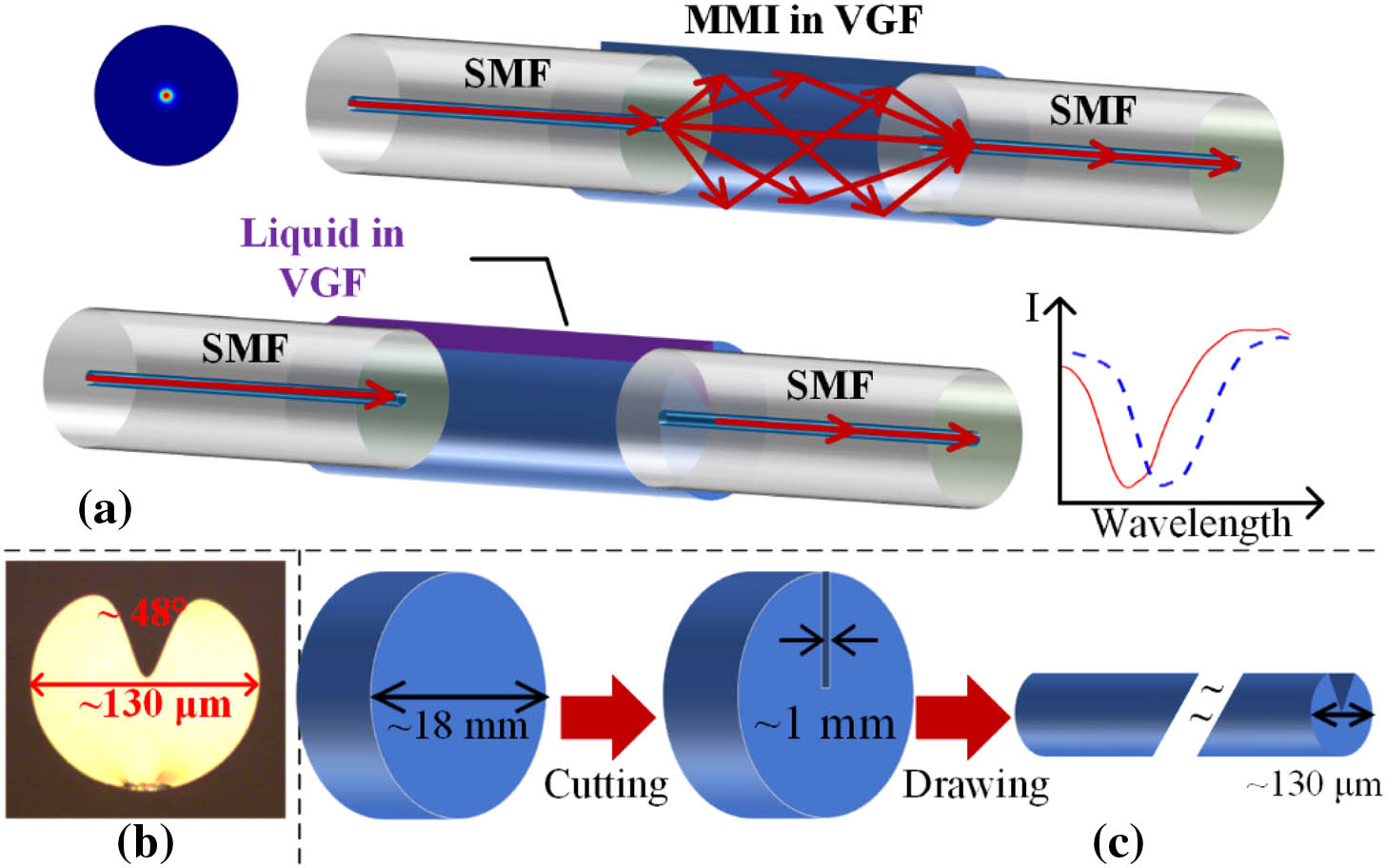
Figure 1.(a) Proposed refractive index sensor structure. The input single-mode fiber (SMF) was fusion spliced to V-groove fiber (VGF) where MMI occurred. The MMI spectrum is transmitted through the output SMF. The liquid droplet was dispensed over the VGF, and the spectral shift caused by the RI change in the surrounding medium was measured. (b) Cross-sectional microphotograph of the fabricated VGF used in the experiments; (c) schematic process to make the VGF.
VGFs can be fabricated in a simple process as schematically shown in Fig. 1(c). A silica rod with diameter of was processed with a diamond saw to inscribe a slot of width that reached near the center of the silica rod. The slotted rod was then drawn to the VGF using an optical fiber drawing tower at an optimal temperature of , and the drawing tension was adjusted to maintain the uniform cross section. The fabricated VGF had an outer diameter of , and the angle of the V-groove was as shown in Fig. 1(b), which can contain hydrophilic liquids such as water and alcohol and their mixture in the nanoliter scale. Note that the groove edge did not reach the center of the VGF in order to excite its fundamental mode and symmetric modes by the LP01 mode from the input SMF, facilitating MMI generation within a short segment of .
2. MODAL ANALYSES OF LIGHT GUIDED THROUGH THE V-GROOVE FIBER
The modes guided along the VGF were identified, and their properties were numerically analyzed using a commercial finite element method (FEM) software, COMSOL. In the simulation, we assumed a VGF structure similar to Fig. 1(b) such that it had a circular outer diameter of 130 μm and the opening angle of the V groove was 48°. The V groove was fitted by a Gaussian-shape function, and its edge was separated by from the VGF center. The cross section of the fiber structure used in the simulation is shown in Fig. 2(a). Here, we assumed the refractive index of the liquid in the V groove is 1.35 and the surrounding medium is the air. The intensity profiles of a few modes guided along the VGF are shown in Figs. 2(b)–2(h). The birefringence of the corresponding HE modes and EH modes is less than . Here the light wavelength was . The refractive index of silica at was assumed to be [35]. Then we calculated the coupling coefficients of the first 50 degenerate modes by using the following equation: where and are the power distribution of the SMF and the th mode of the VGF, respectively. We selected the modes whose coupling coefficients were larger than 1.5%, which are assumed to be excited well in the VGF. The effective RI and the corresponding coefficient are shown in Table 1.
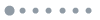
Figure 2.(a) Schematic cross-sectional view of the VGF in simulations; (b)–(h) guide modes along the V-groove fiber and their intensity distribution at . Here we assume the refractive index of liquid to be 1.35.
Effective RI | 1.4439451 | 1.4437885 | 1.4435319 | 1.4434210 | 1.4431758 |
η (%) | 1.57 | 5.53 | 10.06 | 2.38 | 13.13 |
Effective RI | 1.4430183 | 1.4428857 | 1.4427180 | 1.4425125 | – |
η (%) | 4.18 | 1.77 | 10.21 | 5.67 | – |
Table 1. Effective RI and the Corresponding Coefficient of the Excited Modes in VGF
In the previous circular coreless silica fiber (CSF) used in Ref. [23], refractive sensing was only possible by exciting the specific ring-shaped higher-order modes. In contrast, the VGF provided efficient evanescent wave interaction with the liquid, even from the fundamental mode and low-order excited modes as shown in Fig. 2. Therefore, VGF can have an inherently high RI sensitivity to all the dominant guided modes, enabling an efficient RI sensing for the liquid volume of a few tens of nanoliters, which is several orders of magnitude less than prior reports. In Fig. 2, we simulated the mode intensity profiles, assuming the RI of the liquid to be 1.35, which is confined within the groove. We also assumed that the liquid would form the circular outer circumference to make the liquid-filled VGF a cylinder shape, which is consistent with the experimental observations.
We further simulated the impacts of the surrounding medium’s RI on the effective index of the fundamental mode at . First, we changed the refractive index of the surrounding liquid from 1.3 to 1.4, maintaining the liquid circular outer boundary radius at ; the results are summarized in Fig. 3(a). The variation in the of the fundamental mode showed a monotonic and significant increase with , which confirms that even the fundamental mode can directly interact with the surrounding medium by the virtue of the V groove. We then analyzed the impact of the surrounding medium’s volume by varying its radius from 65 to 120 μm; these results are summarized in Fig. 3(b). Note that at the liquid fills only the V groove. For a fixed liquid RI of , the of the fundamental mode showed a very negligible variation only about , which is 20 times smaller than the change caused by the surrounding RI. These results strongly indicate that we can use a VGF as an RI sensor independent of the liquid volume as long as the liquid fills the V groove without using a separate liquid bath as in all prior RI sensors. Note that the obviation of the bulky liquid bath can provide real-time in situ RI sensing using a single drop of liquid, which has not been achieved in prior works.
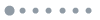
Figure 3.(a) Impacts of on the effective index of the fundamental mode . (b) Impact of the liquid outer boundary radius on the effective index of the fundamental mode .
Light injected from the input SMF will excite the modes in the VGF, which will in turn generate multimode interference. The output spectrum can be expressed as [36] where is the total number of coupled modes in the VGF, is the incident light spectrum, and represents the power coupling between the mode of the input SMF and the th excited mode in the VGF. is the phase difference between the th and th modes of the VGF, which can be expressed as where stands for the effective RI of the th excited mode in the VGF. is the length of the VGF, and is the wavelength of the incident light. When the surrounding RI changes, the spectrum will shift due to , which is the fundamental principle of the proposed RI sensor. In the following sections, fabrication of the proposed sensor and its sensing performance will be discussed.
3. EXPERIMENTAL RESULT
Figure 4 is the schematic diagram for RI sensing experiments using the proposed sensor based on the SMF–VGF–SMF (SVS) structure. The centers of the SMFs and the VGF were aligned manually in a fusion splicer, and the arc current and duration were optimized to keep the V groove intact, whose length was . The amplified spontaneous emission (ASE) light around was launched into the SVS structure, and its output was measured using an optical spectrum analyzer. We prepared an ethanol–water solution with various ethanol concentrations to cover the RI range from 1.33 to 1.37. A single drop of liquid with a volume of nanoliters was directly dispensed onto the V groove using a commercial dispenser. Note that this V-groove filling condition is similar to the simulation mentioned in Fig. 3(c) such that we can neglect the excess volume of liquid in the experiments as long as the V groove is filled.
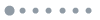
Figure 4.Schematic view of the RI sensing based on an SVS structure. Here ASE is the amplified spontaneous emission light source; OSA is the optical spectrum analyzer. SVS stands for SMF-VGF-SMF structure.
The transmission spectra through the sensor were recorded for various liquids by using an optical spectrum analyzer at room temperature, and the results are summarized in Fig. 5(a). Here we changed the ethanol concentration in the aqueous solution from 0 to 80% to change the RI in a range from 1.33 to 1.37 [37]. Before changing solution with different concentrations, a cleaning process should be done. The cleaning process includes the following steps: (1) remove the liquid in the VGF by using dust-free paper; (2) apply the stream of a solvent consisting of isopropanol and deionized high-purity water over the VGF; (3) apply the stream of deionized high-purity water over the VGF; (4) remove the water in the VGF by using dust-free paper; (5) measure the spectrum of the sensor in the air without filling the liquid and compare it to the reference spectrum to ensure the sensor is clean. Due to the MMI across the VGF, two dips in the transmission spectra were observed near and 1537 nm for the pure water whose refractive index is 1.33. To avoid the distortion problem of the measurement spectra, which may affect the accuracy of minimum detection, a simple moving-average algorithm is used in data processing; see the blue curve in Fig. 5(a). With the increment of the ethanol concentration and subsequent increase in RI, the spectral dips red-shift toward longer wavelengths. We traced only the dip around as an RI sensing spectral indicator because it had a higher visibility than the other dip near . The red-shift of the spectral indicator for various ethanol concentrations in the aqueous solution is summarized in Fig. 5(b) and shows a monotonic increase. In terms of the RI of the solution, the red-shift of the spectral indicator is plotted in Fig. 5(c); it was well fitted by a linear function, and the corresponding RI sensitivity was estimated to be 159.696 nm/RIU in the range of 1.33–1.37, which is lower than that of silica and highly compatible to bio-liquids. Note that the sensitivity of the proposed sensor for a single droplet is comparable to prior MMI sensors [23,24], which used a designated liquid bath. We experimentally confirmed that the proposed SVS structure can provide a high RI sensitivity by directly dispensing a single liquid drop whose volume is in the nanoliter scale, which is several orders of magnitude less than prior reports enabling a real-time in situ RI sensing of bio-liquids.
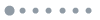
Figure 5.(a) Transmission spectra through the proposed sensor for various ethanol concentrations in the aqueous solution. (b) Shift of the spectral dip for various ethanol concentrations. (c) Spectral shift versus the RI of the solution.
In order to confirm the cross-sensitivity between the temperature and RI, we varied the temperature of the sensing environment from 25°C to 75°C by putting the liquid dispensed SVS structure in a temperature-controlled chamber. This temperature range was deliberately chosen due to its importance in biomedical applications. Once a liquid drop was dispensed, the proposed sensor did not change the transmission spectra in the temperature range, which is consistent with prior sensors that used coreless silica fiber as the sensing medium [23]. We experimentally confirmed that the proposed SVS structure can be readily used as an RI sensor independent of the temperature in the usual biomedical application environment.
4. CONCLUSION
In conclusion, a V-groove fiber (VGF)-based multimode interference liquid refractive-index (RI) sensor was proposed, and the all-fiber structure successfully demonstrated efficient low-RI liquid sensing in the range from to 1.37, which is highly compatible with those of bio-liquids. A segment of VGF with outer diameter and V-groove open angle was serially concatenated between two SMFs to realize an SMF–VGF–SMF (SVS) structure. When a single drop of liquid with a volume of nanoliters was directly dispensed onto the V groove, the transmission spectrum of the SVS monotonically shifted to provide an RI sensitivity of 159.696 nm/RIU. Moreover, due to the all-silica structure, the sensor also showed a negligible temperature cross-sensitivity in the range 25°C–75°C, which overlaps with the biological temperature window. Nanoliter-scale single-liquid-drop RI sensing was achieved, and it can find ample opportunities and potential in real-time in situ applications in biological and medical technologies.
References
[1] P. Vaiano, B. Carotenuto, M. Pisco, A. Ricciardi, G. Quero, M. Consales, A. Crescitelli, E. Esposito, A. Cusano. Lab on fiber technology for biological sensing applications. Laser Photon. Rev., 10, 922-961(2016).
[2] F. Chiavaioli, F. Baldini, S. Tombelli, C. Trono, A. Giannetti. Biosensing with optical fiber gratings. Nanophotonics, 6, 663-679(2017).
[3] C. Park, S. Y. Lee, G. Kim, S. J. Lee, J. Lee, T. Heo, Y. Park, Y. K. Park. Three-dimensional refractive-index distributions of individual angiosperm pollen grains. Curr. Opt. Photon., 2, 460-467(2018).
[4] H.-T. Cho, G.-S. Seo, O.-R. Lim, W. Shin, H.-J. Jang, T.-J. Ahn. Ultraviolet light sensor based on an azobenzene-polymer-capped optical-fiber end. Curr. Opt. Photon., 2, 303-307(2018).
[5] A. Kumar, T. Subrahmanyam, A. Sharma, K. Thyagarajan, B. Pal, I. Goyal. Novel refractometer using a tapered optical fibre. Electron. Lett., 20, 534-535(1984).
[6] O. Frazão, T. Martynkien, J. Baptista, J. Santos, W. Urbanczyk, J. Wojcik. Optical refractometer based on a birefringent Bragg grating written in an H-shaped fiber. Opt. Lett., 34, 76-78(2009).
[7] S.-M. Lee, S. S. Saini, M.-Y. Jeong. Simultaneous measurement of refractive index, temperature, and strain using etched-core fiber Bragg grating sensors. IEEE Photon. Technol. Lett., 22, 1431-1433(2010).
[8] C.-L. Zhao, L. Qi, S. Zhang, Y. Jin, S. Jin. Simultaneous measurement of refractive index and temperature based on a partial cone-shaped FBG. Sens. Actuators B Chem., 178, 96-100(2013).
[9] G. Laffont, P. Ferdinand. Tilted short-period fibre-Bragg-grating-induced coupling to cladding modes for accurate refractometry. Meas. Sci. Technol., 12, 765-770(2001).
[10] T. Guo, H.-Y. Tam, P. A. Krug, J. Albert. Reflective tilted fiber Bragg grating refractometer based on strong cladding to core recoupling. Opt. Express, 17, 5736-5742(2009).
[11] T. Wang, K. Liu, J. Jiang, M. Xue, P. Chang, T. Liu. Temperature-insensitive refractive index sensor based on tilted moiré FBG with high resolution. Opt. Express, 25, 14900-14909(2017).
[12] I. M. Ishaq, A. Quintela, S. W. James, G. J. Ashwell, J. M. Lopez-Higuera, R. P. Tatam. Modification of the refractive index response of long period gratings using thin film overlays. Sens. Actuators B Chem., 107, 738-741(2005).
[13] C. Guan, X. Tian, S. Li, X. Zhong, J. Shi, L. Yuan. Long period fiber grating and high sensitivity refractive index sensor based on hollow eccentric optical fiber. Sens. Actuators B Chem., 188, 768-771(2013).
[14] T. Geng, S. Zhang, F. Peng, W. Yang, C. Sun, X. Chen, Y. Zhou, Q. Hu, L. Yuan. A temperature-insensitive refractive index sensor based on no-core fiber embedded long period grating. J. Lightwave Technol., 35, 5391-5396(2017).
[15] T. Yadav, R. Narayanaswamy, M. A. Bakar, Y. M. Kamil, M. Mahdi. Single mode tapered fiber-optic interferometer based refractive index sensor and its application to protein sensing. Opt. Express, 22, 22802-22807(2014).
[16] Y. Zhao, L. Cai, X.-G. Li. In-fiber Mach-Zehnder interferometer based on up-taper fiber structure with Er3+ doped fiber ring laser. J. Lightwave Technol., 34, 3475-3481(2016).
[17] D. W. Kim, F. Shen, X. Chen, A. Wang. Simultaneous measurement of refractive index and temperature based on a reflection-mode long-period grating and an intrinsic Fabry-Perot interferometer sensor. Opt. Lett., 30, 3000-3002(2005).
[18] R. St-Gelais, J. Masson, Y.-A. Peter. All-silicon integrated Fabry-Pérot cavity for volume refractive index measurement in microfluidic systems. Appl. Phys. Lett., 94, 243905(2009).
[19] D. Wu, T. Zhu, G.-Y. Wang, J.-Y. Fu, X.-G. Lin, G.-L. Gou. Intrinsic fiber-optic Fabry-Perot interferometer based on arc discharge and single-mode fiber. Appl. Opt., 52, 2670-2675(2013).
[20] J. Wo, G. Wang, Y. Cui, Q. Sun, R. Liang, P. P. Shum, D. Liu. Refractive index sensor using microfiber-based Mach-Zehnder interferometer. Opt. Lett., 37, 67-69(2012).
[21] H. Luo, Q. Sun, Z. Xu, D. Liu, L. Zhang. Simultaneous measurement of refractive index and temperature using multimode microfiber-based dual Mach-Zehnder interferometer. Opt. Lett., 39, 4049-4052(2014).
[22] Q. Wang, G. Farrell. All-fiber multimode-interference-based refractometer sensor: proposal and design. Opt. Lett., 31, 317-319(2006).
[23] Y. Jung, S. Kim, D. Lee, K. Oh. Compact three segmented multimode fibre modal interferometer for high sensitivity refractive-index measurement. Meas. Sci. Technol., 17, 1129-1133(2006).
[24] M. Park, S. Lee, W. Ha, D. K. Kim, W. Shin, I. B. Sohn, K. Oh. Ultracompact intrinsic micro air-cavity fiber Mach-Zehnder interferometer. IEEE Photon. Technol. Lett., 21, 1027-1029(2009).
[25] M. Iga, A. Seki, K. Watanabe. Gold thickness dependence of SPR-based hetero-core structured optical fiber sensor. Sens. Actuators B Chem., 106, 363-368(2005).
[26] N. Cennamo, D. Massarotti, L. Conte, L. Zeni. Low cost sensors based on SPR in a plastic optical fiber for biosensor implementation. Sensors, 11, 11752-11760(2011).
[27] B.-H. Liu, Y.-X. Jiang, X.-S. Zhu, X.-L. Tang, Y.-W. Shi. Hollow fiber surface plasmon resonance sensor for the detection of liquid with high refractive index. Opt. Express, 21, 32349-32357(2013).
[28] E. Klantsataya, A. François, H. Ebendorff-Heidepriem, P. Hoffmann, T. M. Monro. Surface plasmon scattering in exposed core optical fiber for enhanced resolution refractive index sensing. Sensors, 15, 25090-25102(2015).
[29] J. S. Velázquez-González, D. Monzón-Hernández, D. Moreno-Hernández, F. Martínez-Piñón, I. Hernández-Romano. Simultaneous measurement of refractive index and temperature using a SPR-based fiber optic sensor. Sens. Actuators B Chem., 242, 912-920(2017).
[30] Y. Lin, Y. Zou, Y. Mo, J. Guo, R. G. Lindquist. E-beam patterned gold nanodot arrays on optical fiber tips for localized surface plasmon resonance biochemical sensing. Sensors, 10, 9397-9406(2010).
[31] Y. Shao, S. Xu, X. Zheng, Y. Wang, W. Xu. Optical fiber LSPR biosensor prepared by gold nanoparticle assembly on polyelectrolyte multilayer. Sensors, 10, 3585-3596(2010).
[32] H. Nguyen, F. Sidiroglou, S. Collins, T. Davis, A. Roberts, G. Baxter. A localized surface plasmon resonance-based optical fiber sensor with sub-wavelength apertures. Appl. Phys. Lett., 103, 193116(2013).
[33] J. Chen, S. Shi, R. Su, W. Qi, R. Huang, M. Wang, L. Wang, Z. He. Optimization and application of reflective LSPR optical fiber biosensors based on silver nanoparticles. Sensors, 15, 12205-12217(2015).
[34] T. Nazari, B. Joo, J.-H. Hwang, B. Paulson, J. Park, Y. M. Jhon, K. Oh. Highly birefringent V-groove liquid core fiber. Opt. Express, 25, 24714-24726(2017).
[35] K. Oh, U. C. Paek. Silica Optical Fiber Technology for Devices and Components: Design, Fabrication, and International Standards(2012).
[36] J. Zhang, W. Sun, L. Yuan, G.-D. Peng. Design of a single-multimode-single-mode filter demodulator for fiber Bragg grating sensors assisted by mode observation. Appl. Opt., 48, 5642-5646(2009).
[37] M. T. Tavassoly, A. Saber. Optical refractometry based on Fresnel diffraction from a phase wedge. Opt. Lett., 35, 3679-3681(2010).