
- Photonics Research
- Vol. 9, Issue 10, 2095 (2021)
Abstract
1. INTRODUCTION
One of the most fundamental manipulations of light is its focusing/gathering to a desired location in space [1]. However, optical aberrations, both monochromatic and chromatic, still represent a major hurdle in this endeavor [2]. Chromatic aberration is the phenomenon where the dependence of the material refractive index on wavelength causes the light of different wavelengths to focus on different points in space [3,4]. The creative endeavors to reduce chromatic dispersion started with achromatic doublets in the 18th century [5], followed by applying hybrid refractive–diffractive lenses [6,7] and graded-index optics [8].
In addition, contemporary studies have presented several solutions to overcome chromatic dispersion. The most common methods to design meta-lenses are tailoring the phase profiles [9–13], combining meta-surfaces with refractive devices as a hybrid structure to balance the material dispersion [14], manipulating the polarization rotation of incident light [15], and meta-surfaces doublets [16]. Additionally, multilevel diffractive lenses (MDLs) provide not only achromatic focusing, but also polarization-insensitive, broadband, and energy efficient focusing with their reduced weight and size compared to the meta-lenses [17–21]. In the MDL structures, each zone (concentric ring) plays an important role in reducing chromatic aberration provided that the zone’s parameters are adjusted properly [22]. Hence, various optimization and inverse design methods have been exploited to obtain appropriate MDL parameters for efficient focusing [23–26]. One of the important design characteristics of meta-lenses and MDLs is their numerical aperture (NA) value, which is a function of focal length, the radius of the lens, and the refractive index of the medium. It is difficult to obtain both a high NA value and chromatic aberration-free focusing [27]; however, an appropriate MDL design can overcome low efficiency and chromatic dispersion problems of the meta-lenses [28,29].
In this paper, we present a high-NA, diffraction-limited broadband achromatic multilevel diffractive lens (AMDL) operating in the microwave regime between 10 and 14 GHz. A multi-objective differential evolution (MO-DE) algorithm is applied to minimize chromatic aberration and maximize the AMDL focusing efficiency by tightly confining the diffracted light energy in the main lobe and suppressing the side lobes (suppression of the side lobes is aimed at achieving a clear and efficient focal spot). Here, the heights and the widths of each AMDL zone are optimized to achieve a predefined objective function. Moreover, differently from the proposed achromatic meta-lens and MDL designs in the literature, in this study, the focal distance is directly optimized by the algorithm without fixing it to the desired center frequency (fixing of the center frequency is an important requirement for phase profile engineering in achromatic lens designs). In other words, this approach diminishes the necessity of determining focal distance and center wavelength and provides an alternative way to engineer phase profiles. In addition, to experimentally verify the numerical results, measurements in the microwave regime were performed by fabricating proposed AMDL using 3D-fused deposition modelling (3D-FDM) with polylactic acid (PLA) material. We also scaled down the optimized AMDL to the visible wavelengths by taking 550 nm as a reference wavelength (
Sign up for Photonics Research TOC. Get the latest issue of Photonics Research delivered right to you!Sign up now
2. DESIGN METHODOLOGY AND NUMERICAL RESULTS OF AMDL
In this study, the differential evolution (DE) algorithm is used to obtain an ultra-compact, high-NA and broadband functional achromatic MDL. DE is a metaheuristic approach that provides sufficiently appropriate solutions to multi-objective optimization problems by taking advantage of the biological mechanisms such as cross-over, mutation, and selection of natural evolution process [30]. It is important to note that in our previous works, we have successfully applied the DE algorithm in various photonic and optic applications [4,31–33]. The DE algorithm iteratively converges to a desired target, which is defined as a cost function. The searching ability of DE depends on the defined heuristic mechanism and objective function. Initially, the optimization algorithm starts with a fixed number (
Before the population can be initialized, both upper (
Following the algorithm initialization, i.e., after initial population is generated, we select three different individuals from the instant generation where one of them is the best individual of the corresponding generation. These three vectors are used to create one mutated individual, which is defined as mutation mechanisms. The mutation mechanisms are implemented to generate
The MO-DE optimization algorithm is embedded into a three-dimensional (3D) finite-difference time domain (FDTD) [34] method to design an ultra-compact, high-NA and achromatic MDL. Figure 1(a) illustrates the AMDL structure intended for design with its structural parameters [i.e., heights (
Figure 1.(a) Schematic representation of planned AMDL with light-focusing behavior and optimization parameters. (b) Perspective view of designed AMDL consisting of PLA and (c) the quarter-cross sectional view of the lens with the plot of height and width for each ring.
We also note that one can use our approach to design an achromatic lens with the fixed focal length. However, optimizing the focal length value plays a crucial role in the mitigation of chromatic aberration, especially when intended lens design has limits on the geometrical parameters due to available fabrication and characterization facilities, like in the case of proposed AMDL. Optimizing focal distance becomes more important if the design approach is based on heuristics rather than exact analytical design, like in the case of conventional diffractive lenses. In our opinion, proposed design approach, where focal distance is not fixed but optimized, is beneficial for improving the achromatic performance of the MDL structures.
The designed lens is composed of PLA material, which has a low permittivity value
The MO-DE minimizes
Figure 2 shows the numerically calculated steady-state spatial electric field intensity (
Figure 2.Numerically calculated electric field intensity distributions (top) and calculated intensity distributions around the focal points with their lateral cross-sectional profiles and corresponding FWHM and MSLL values (bottom) at (a) 10 GHz, (b) 11 GHz, (c) 12 GHz, (d) 13 GHz, and (e) 14 GHz. The white horizontal dashed line indicates the desired focal distance (
To analyze the broadband operation and performance of the designed AMDL, Fig. 3 was prepared. The maps of cross-sectional electric field intensity (
Figure 3.Map of cross-sectional electric field intensity distributions in (a) longitudinal direction on the optical axis and (b) lateral direction at focal points. The black vertical dashed line represents a desired focal distance (
There is a smooth transition from the general trend (being achromatic) to a wavelength-dependent focusing at around 12.5 GHz. Both the focal point and FWHM reach minimum values at this frequency, and the achromatic response is apparent as we continue tracing the rest of the bandwidth. It is surprising that the deployed algorithm generates an AMDL holding four zones and binary gratings of different heights and widths in a rather regular fashion. However, some of the structural parameters break the design rules of diffractive lens that can be used based on the analytical expressions [22]. Hence, even though it is difficult to identify the exact physical mechanism behind the characteristic observed at around 12.5 GHz, this behavior could be attributed to weakly guided resonance mode effect. When we calculated the transmission coefficient (plot skipped for simplicity), we observed a slight dip in the transmitted light (at exactly 12.68 GHz). It could be an indication of the light coupling to weakly guided mode in AMDL. By inspecting the field plots in the
In Fig. 3(c), calculated focal distances (
It is also important to quantitively analyze the broadband focusing performance of the proposed AMDL. For this purpose, the focusing efficiency and NA values were extracted and demonstrated in Fig. 3(d). Within the defined frequency interval, NA values are calculated as
It is important to note that the Strehl ratio is more relevant performance criteria than presenting the resolution in terms of FWHM [44]. For this reason, we calculated the Strehl ratio for the proposed AMDL, and an average Strehl ratio value emerged as 0.7174 for an operating frequency region between 10 and 14 GHz.
3. EXPERIMENTAL ANALYSIS OF ACHROMATIC FOCUSING EFFECT
To verify the operation principle and focusing performance of the designed AMDL, experiments were conducted in the microwave frequency region. The designed lens was produced using 3D–FDM with PLA thermoplastic polyester material. PLA is an all-dielectric, affordable, and biodegradable material that has a low dielectric constant value of
The photographic illustrations of the perspective and top views of the fabricated AMDL are presented in Figs. 4(a) and 4(b), respectively. Furthermore, the stand for the AMDL to allocate it straight [in parallel to the horn antenna that illuminates AMDL with microwave source, see Fig. 4(a)] is produced and is covered by microwave-absorbing materials to reduce the undesirable reflections. In the microwave experiments, an Agilent E5071C ENA vector network analyzer (VNA) was used to produce Gaussian profiled electromagnetic wave source through a horn antenna connected to VNA, whereas a monopole antenna was employed to scan the electric field distributions. The photographic illustration of the experimental setup and schematic view of the experimental measurements are presented in Figs. 4(c) and 4(d), respectively. In Fig. 4(d), a schematic of electric field distribution scanning areas is presented and defined as Scanning Area I for the
Figure 4.Photograph of the 3D printed AMDL from (a) perspective and (b) top views. (c) Photographic view of the experimental setup. (d) Schematic representation of experimental setup to scan electric field intensity distributions at back focal plane (
Experimentally measured electric field distributions at the focal plane (
To verify broadband operation functionality of the designed AMDL, the maps of the experimentally measured cross-sectional intensity profiles on the optical axis and on the lateral direction of focal point are presented in Figs. 5(a) and 5(b), respectively. The similarity in the numerical calculations and experimental measurements is evident, except for small shifts in the
Figure 5.Maps of experimentally measured cross-sectional intensity profiles (a) on the optical axis and (b) at focal points. The black vertical dashed line represents desired focal distance (
It is important to note that the similar effect of a weakly guided resonance mode was not observed in the experimental results, as can be noticed in Fig. 5(a) (it is also evident in the zoom-out region that is given as an inset). For this reason, we prepared Fig. 5(d) to inspect in detail the focal shifts between 12 and 13 GHz. If we look at the extracted back focal distance values between 12 and 13 GHz presented in Fig. 5(d), we can see the abrupt deviation from the average focal distance starting from 12.4 GHz (shaded regions in the plot are given to emphasize the envelope of focal shift variations). In addition, there is a sharp deep in focal distance values between 12.5 and 12.7 GHz. This deviation and sharp deep in focal distances could be a sign of a weak resonance effect. However, we should note that these observations do not guarantee that we have the same resonance effect as in numerical results. In our opinion, a resonance effect may not be observed because of the possible slight difference in refractive indices of the AMDL material for simulations and experiments. Moreover, it is also impossible to meet the ideal computational domain conditions in experimental measurements like in simulations (presence of possible back-reflections and parasitic scatterings from the experimental environment).
It is difficult to directly measure the focusing efficiency of the AMDL through our microwave experimental setup; hence, we used an indirect method that resembles the numerical calculation [38,39] of the focusing efficiency of fabricated lens to provide comparable data. Here, the transmission value falling into a circular aperture with a radius equaling three times the FWHM centered at focal point is a proportioned transmission value falling into whole focal plane (
Numerical and Experimental Characteristics of AMDL with Their Average Values
Frequency (GHz) | 3D FDTD | Experiment | ||||||||
---|---|---|---|---|---|---|---|---|---|---|
FWHM ( | MSLL (n.i.) | NA | F.E. (%) | FWHM ( | MSLL (n.i.) | NA | F.E. (%) | |||
9.92 | 0.423 | 0.253 | 0.994 | 45.55 | 7.52 | 0.562 | 0.351 | 0.997 | 32.19 | |
13.27 | 0.508 | 0.192 | 0.990 | 38.23 | 10.57 | 0.635 | 0.382 | 0.994 | 30.14 | |
14.42 | 0.554 | 0.168 | 0.988 | 39.94 | 11.47 | 0.590 | 0.275 | 0.992 | 26.03 | |
14.42 | 0.550 | 0.105 | 0.988 | 35.41 | 19.65 | 0.726 | 0.230 | 0.979 | 23.18 | |
14.42 | 0.485 | 0.078 | 0.988 | 27.91 | 16.96 | 0.575 | 0.277 | 0.983 | 22.35 | |
13.29 | 0.504 | 0.159 | 0.990 | 37.41 | 13.23 | 0.618 | 0.303 | 0.989 | 26.78 |
n.i., normalized intensity.
To compare the results of numerical calculation and experimental measurements, all data are collected in Table 1, and we can conclude that the experimental and numerical results are in good agreement with each other. Considering the average data (last column in Table 1), the NA values are almost the same in both cases. The differences in other parameters are within the acceptable ranges and might be caused by problems such as exciting the structure with a partial plane wave due to finite aperture size of the horn antenna and the misalignment of the antenna with the AMDL.
4. DISCUSSION
It is instructive to inspect the focusing performance of our optimized AMDL in the visible region. Unfortunately, due to the absence of an experimental facility, we could not carry out our analysis experimentally. Instead, we scaled the structural parameters of our designed AMDL to visible wavelengths to analyze its performance in the visible regime.
For this purpose, we chose 550 nm as a reference wavelength (
Figure 6.(a) Quarter-cross sectional view of the nano-scaled lens with the plot of height and width for each zone. (b) Map of cross-sectional intensity profiles on optical axis, (c) plots of both Δ
The electric field intensity (
Figure 7.Numerically calculated electric field intensity distributions with 40-nm-wavelength steps at (a) 380 nm, (b) 420 nm, (c) 460 nm, (d) 500 nm, (e) 540 nm, (f) 580 nm, and (g) 620 nm. The dashed lines represent the average focal distance (233 nm).
5. CONCLUSION
This study demonstrated an all-dielectric achromatic MDL design operating between 10 and 14 GHz. The structural parameters of each AMDL zone were optimized through the 3D FDTD method, combined with a multi-objective differential evolution (MO-DE) algorithm. The optimized AMDL was fabricated via 3D-printing by using a low refractive index PLA (
Acknowledgment
Acknowledgment. H. Kurt acknowledges partial support from the Turkish Academy of Sciences. Authors would like to thank Jacob Engelberg from The Hebrew University of Jerusalem for his valuable comments and suggestions. H. Kurt acknowledges the financial support of KAIST Startup Funding (Project number G04200054).
References
[1] R. G. Gonzáles-Acuña, H. A. Chaparro-Romo, J. C. Gutiérrez-Vega. Analytical Lens Design(2020).
[2] E. L. Dereniak, T. D. Dereniak. Aberrations in optical systems. Geometrical and Trigonometric Optics, 292-322(2008).
[3] M. Born, E. Wolf. Principles of Optics(1980).
[4] B. K. Yildirim, E. Bor, H. Kurt, M. Turduev. Zones optimized multilevel diffractive lens for polarization-insensitive light focusing. J. Phys. D, 53, 495109(2020).
[5] R. Willach. New light on the invention of the achromatic telescope objective. Notes Rec. R. Soc. Lond., 50, 195-210(1996).
[6] W. Harm, C. Roider, A. Jesacher, S. Bernet, M. Ritsch-Marte. Dispersion tuning with a varifocal diffractive-refractive hybrid lens. Opt. Express, 22, 5260-5269(2014).
[7] S. Gorelick, A. de Marco. Hybrid refractive-diffractive microlenses in glass by focused Xe ion beam. J. Vac. Sci. Technol. B, 37, 051601(2019).
[8] R. Buczynski, A. Filipkowski, B. Piechal, H. T. Nguyen, D. Pysz, R. Stepien, A. Waddie, M. R. Taghizadeh, M. Klimczak, R. Kasztelanic. Achromatic nanostructured gradient index microlenses. Opt. Express, 27, 9588-9600(2019).
[9] F. Aieta, M. A. Kats, P. Genevet, F. Capasso. Multiwavelength achromatic metasurfaces by dispersive phase compensation. Science, 347, 1342-1345(2015).
[10] M. Khorasaninejad, Z. Shi, A. Y. Zhu, W. T. Chen, V. Sanjeev, A. Zaidi, F. Capasso. Achromatic metalens over 60 nm bandwidth in the visible and metalens with reverse chromatic dispersion. Nano Lett., 17, 1819-1824(2017).
[11] W. T. Chen, A. Y. Zhu, V. Sanjeev, M. Khorasaninejad, Z. Shi, E. Lee, F. Capasso. A broadband achromatic metalens for focusing and imaging in the visible. Nat. Nanotechnol., 13, 220-226(2018).
[12] Q. Cheng, M. Ma, D. Yu, Z. Shen, J. Xie, J. Wang, N. Xu, H. Guo, W. Hu, S. Wang, T. Li, S. Zhuang. Broadband achromatic metalens in terahertz regime. Sci. Bull., 64, 1525-1531(2019).
[13] S. Shrestha, A. C. Overvig, M. Lu, A. Stein, N. Yu. Broadband achromatic dielectric metalenses. Light Sci. Appl., 7, 85(2018).
[14] R. Sawant, P. Bhumkar, A. Y. Zhu, P. Ni, F. Capasso, P. Genevet. Mitigating chromatic dispersion with hybrid optical metasurfaces. Adv. Mater., 31, 1805555(2018).
[15] M. D. Aiello, A. S. Backer, A. J. Sapon, J. Smits, J. D. Perreault, P. Llull, V. M. Acosta. Achromatic varifocal metalens for the visible spectrum. ACS Photon., 6, 2432-2440(2019).
[16] D. Tang, L. Chen, J. Liu, X. Zhang. Achromatic metasurface doublet with a wide incident angle for light focusing. Opt. Express, 28, 12209-12218(2020).
[17] P. Wang, N. Mohammad, R. Menon. Chromatic-aberration-corrected diffractive lenses for ultrabroadband focusing. Sci. Rep., 6, 21545(2016).
[18] N. Mohammad, M. Meem, B. Shen, P. Wang, R. Menon. Broadband imaging with one planar diffractive lens. Sci. Rep., 8, 2799(2018).
[19] S. Banerji, M. Meem, A. Majumder, C. Dvonch, B. Sensale-Rodriguez, R. Menon. Single flat lens enabling imaging in the short-wave infra-red (SWIR) band. OSA Contin., 2, 2968-2974(2019).
[20] S. Banerji, M. Meem, A. Majumder, F. G. Vasquez, B. Sensale-Rodriguez, R. Menon. Imaging with flat optics: metalenses or diffractive lenses?. Optica, 6, 10-805(2019).
[21] S. Wang, P. C. Wu, V. C. Su, Y. C. Lai, M. K. Chen, H. Y. Kuo, B. H. Chen, Y. H. Chen, T. T. Huang, J. H. Wang, R. M. Lin, C. H. Kuan, T. Li, Z. Wang, S. Zhu, D. P. Tsai. A broadband achromatic metalens in the visible. Nat. Nanotechnol., 13, 227-232(2018).
[22] D. C. O’Shea, T. J. Suleski, A. D. Kathman, D. W. Prather. Diffractive Optics: Design, Fabrication, and Test(2003).
[23] E. Noponen, J. Turunen, A. Vasara. Parametric optimization of multilevel diffractive optical elements by electromagnetic theory. Appl. Opt., 31, 5910-5912(1992).
[24] M. Meem, S. Banerji, A. Majumder, F. G. Vasquez, B. Sensale-Rodriguez, R. Menon. Broadband lightweight flat lenses for long-wave infrared imaging. Proc. Natl. Acad. Sci. USA, 116, 21375-21378(2019).
[25] S. Banerji, B. Sensale-Rodriguez. Inverse designed achromatic flat lens operating in the ultraviolet. OSA Contin., 3, 1917-1929(2020).
[26] M. Meem, S. Banerji, A. Majumder, C. Pies, T. Oberbiermann, B. Sensale-Rodriguez, R. Menon. Inverse-designed achromatic flat lens enabling imaging across the visible and near-infrared with diameter > 3 mm and NA = 0.3. Appl. Phys. Lett., 117, 041101(2020).
[27] H. Liang, A. Martins, B. V. Borges, J. Zhou, E. R. Martins, J. Li, T. F. Krauss. High performance metalenses: numerical aperture, aberrations, chromaticity, and trade-offs. Optica, 6, 1461-1470(2019).
[28] M. Meem, S. Banerji, C. Pies, T. Oberbiermann, A. Majumder, B. Sensale-Rodriguez, R. Menon. Large-area, high-numerical-aperture multi-level diffractive lens via inverse design. Optica, 7, 252-253(2020).
[29] H. Chung, O. D. Miller. High-NA achromatic metalenses by inverse design. Opt. Express, 28, 6945-6965(2020).
[30] K. V. Price, R. M. Storn, J. A. LaMoine. Differential Evolution: A Practical Approach to Global Optimization, 539(2005).
[31] B. Neseli, E. Bor, H. Kurt, M. Turduev. Rainbow trapping in a tapered photonic crystal waveguide and its application in wavelength demultiplexing effect. J. Opt. Soc. Am. B, 37, 1249-1256(2020).
[32] E. Bor, H. Kurt, M. Turduev. Metaheuristic approach enabled mode order conversion in photonic crystals: numerical design and experimental realization. J. Opt., 21, 085801(2019).
[33] E. Bor, M. Turduev, U. G. Yasa, H. Kurt, K. Staliunas. Asymmetric light transmission effect based on an evolutionary optimized semi-dirac cone dispersion photonic structure. Phys. Rev. B, 98, 245112(2018).
[34] http://www.lumerical.com/tcad-products/fdtd/. http://www.lumerical.com/tcad-products/fdtd/
[35] W. B. Weir. Automatic measurement of complex dielectric constant and permeability at microwave frequencies. Proc. IEEE, 62, 33-36(1974).
[36] D. M. Abdalhadi, Z. Abbas, A. F. Ahmad, K. A. Matori, F. Esa. Controlling the properties of OPEFB/PLA polymer composite by using Fe2O3 for microwave applications. Fibers Polym., 19, 1513-1521(2018).
[37] J. P. Berenger. A perfectly matched layer for the absorption of electromagnetic waves. J. Comput. Phys., 114, 185-200(1994).
[38] A. Arbabi, Y. Horie, A. J. Ball, M. Bagheri, A. Faraon. Subwavelength-thick lenses with high numerical apertures and large efficiency based on high-contrast transmitarrays. Nat. Commun., 6, 7069(2015).
[39] N. Yilmaz, A. Ozer, A. Ozdemir, H. Kurt. Nano-hole based phase gradient metasurfaces for light manipulation. J. Phys. D, 52, 205102(2019).
[40] G. J. Swanson. Binary optics technology: theoretical limits on the diffraction efficiency of multilevel diffractive optical elements(1989).
[41] M. Khorasaninejad, F. Capasso. Metalenses: versatile multifunctional photonic components. Science, 358, eaam8100(2017).
[42] S. Banerji, B. Sensale-Rodriguez. A computational design framework for efficient, fabrication error-tolerant, planar THz diffractive optical elements. Sci. Rep., 9, 5801(2019).
[43] P. Lalanne, P. Chavel. Metalenses at visible wavelengths: past, present, perspectives. Laser Photon. Rev., 11, 1600295(2017).
[44] J. Engelberg, U. Levy. Achromatic flat lens performance limits. Optica, 8, 834-845(2021).
[45] C. M. B. Gonçalves, J. A. P. Coutinho, I. M. Marrucho. Poly-(Lactic Acid): Synthesis, Structures, Properties, Processing, and Applications, 97-112(2010).
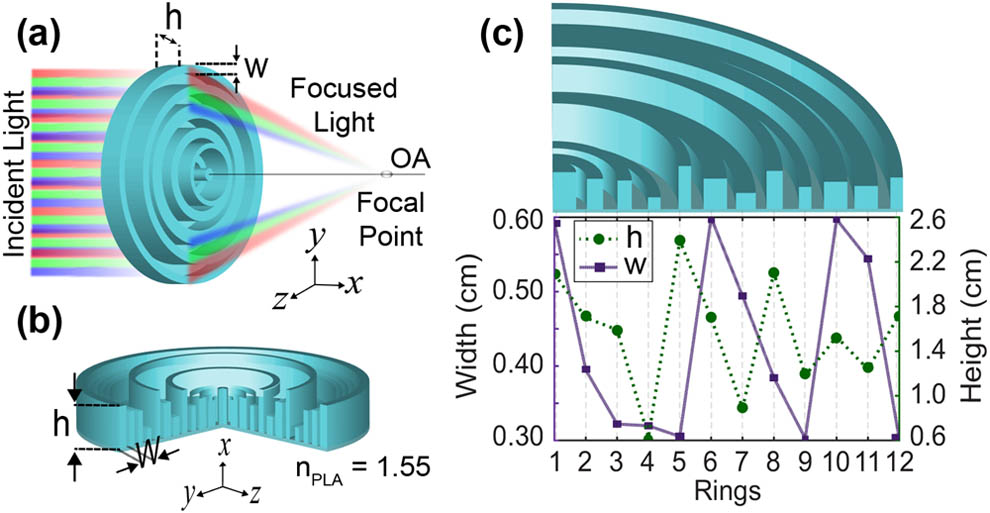
Set citation alerts for the article
Please enter your email address