Abstract
We demonstrate a potassium titanyl phosphate-based optical parametric oscillator (OPO) emitting at 1729 nm. A maximum output power of 1.56 W at 1729.4 nm is obtained with an original fundamental laser power of 5.48 W. The pulse with a pulse duration of 11.22 ns exceeds 3 mJ at a 500 Hz repetition rate. To our knowledge this is the highest energy output of an OPO laser emitting around 1.73 μm operating at a 100 Hz order of magnitude. This laser is primarily used for bond-selective imaging of deep tissue, a promising way for diagnosing vulnerable plaques in live patients.The generation of lasers radiating in the infrared has attracted great interest for their important applications in medical diagnostics, food and agrochemical quality control, neuroimaging, etc.[1–4]. Recent research reveals the existence of an optical window between 1600 and 1850 nm that makes it promising for deep tissue imaging. In this optical window lasers radiating around 1730 nm play an important role for intravascular photoacoustic imaging, a well-established, hybrid, and most promising candidate for noninvasive measurement techniques[5]. High repetition rate nanosecond lasers around 1730 nm currently are applied to this technique. However, this imaging technique has been stifled by its slow imaging speed, which mainly depends on the repetition rate of the pulse[6]. As higher pulse repetition will make the imaging system work faster, this tissue imaging technique calls for a laser emitting around 1730 nm with a high pulse repetition rate.
Traditionally it is possible to obtain a laser emitting at 1730 nm directly by pumping an Er:YLF crystal[7]. Doroshenko et al. have already adopted a flashlamp-pumped Er:YLF laser with a quasi-continuous output of more than 800 mJ[8]. Barnes et al. have reported a Q-switched Er:YLF laser with a pulse energy of 25 mJ pumped by a flashlamp. The repetition of the pulse in the system is limited to 5 Hz. The short wavelength pump bands of Er:YLF cannot match the commercial laser diode (LD) emitting waveband, so it is not possible to pump the crystal with a LD[9]. This leads to low power conversion efficiency. Moreover, higher repetition rates bring a serious thermal effect while pumped by a Xe flashlamp. Being pumped by a flashlamp also leads to low power conversion efficiency. Therefore, it is intractable to obtain a laser emitting around 1730 nm at a high repetition rate by using an Er:YLF crystal.
Another way to obtain a 1.73 μm laser is with an optical parametric oscillator (OPO). In the past few years interest in efficient eye-safe lasers has stimulated the development of OPOs[10–12]. Since 1995, quasi-phase-matching (QPM) schemes were also popularly used to obtain infrared laser radiation[13]. This inspired us to make an OPO matching the demand. Many tunable OPOs already have a tuning range including 1730 nm. In 2005, Peng et al. reported a high-repetition-rate tunable OPO with a wide tuning range from 1650 to 2100 nm[14]. Only about 1.5 mJ can be obtained at 1730 nm. Even some commercial OPO enterprises such as OPOTEK also have several tunable products with a tuning range including 1730 nm. However, either their tunable product operating repetition rate stays in a ten hertz order of magnitude, such as Opolette 532, or the output energy of their products is not strong enough for an imaging system such as Opolette HR. Consequently, these products cannot solve the problem of accelerating the imaging speed. In order to solve the problem, a high-power laser working at a high repetition rate emitting around 1.73 μm needs to be developed.
Sign up for Chinese Optics Letters TOC. Get the latest issue of Chinese Optics Letters delivered right to you!Sign up now
Here, a potassium titanyl phosphate (KTP)-OPO driven by a diode-end-pumped Nd:YAG laser emitting at around 1.73 μm is demonstrated for the first time to our knowledge. Under a pumping laser power of 5.48 W and repetition rate of 500 Hz, a maximum average output power of 1.56 W at 1729.4 nm was obtained, corresponding to an optical-optical conversion efficiency of 28.5% from an original fundamental laser to a signal laser. A pulse energy of more than 3 mJ at 500 Hz with a pulse duration of 11.22 ns was obtained. The output pulse energy fluctuation supervised by a power meter of 1.73 μm was less than 0.5% during the 2 h operating period.
A schematic diagram of the experimental setup is depicted in Fig. 1. Two fiber-coupled LDs with a 30 W maximum output power emitting at 808 nm were used as the pump source. The fiber core diameter was 0.6 mm. The pump light was inserted into the laser crystal by two groups of 2:5 coupling lenses at the same time. A 0.3 at. %-doped composite Nd:YAG crystal with a diameter of 4 mm and length of 40 mm was adopted as the active medium. Considering that we have used double-end pumping in this system, a longer length promises the safety of the LD without being damaged by an unabsorbed diode laser. The crystal also had a 5 mm-long undoped part on both end faces of the crystal while being antireflection (AR) coated at both 808 and 1064 nm. Here we use a composite crystal of low doping mainly to reduce the thermal lens effect to achieve a high-beam-quality original fundamental laser because good beam quality of the laser will improve the conversion efficiency. A plane-plane resonate cavity containing mirrors M1 and M2 were adopted. The flat mirror M1 was high-reflection (HR) coated at 1064 nm. The output mirror M2 was coated with a transmittance equivalent to 60% at 1064 nm. A couple of fold mirrors to complete two-end pumping were AR coated at 808 nm and HR coated at 1064 nm. The E-O Q-switch was composed of a polarizer, a quarter-wave plate (P1) and a KD*P Pockels cell. The pumping pulse duration was 230 ms, just matching closely with the upper laser level lifetime of a Nd:YAG crystal. M1 and M2 mirrors constitute the 1064 nm laser resonate cavity. A pulse of 11 mJ at 1064 nm was obtained with good beam quality. The parametric oscillator was composed of flat mirrors M3 and M4. Both of them were made of quartz for the sake of avoiding absorbing the signal laser. M3 was HR coated at 1730 nm and AR coated at 1064 nm. M4 was AR coated at 1064 nm and had partial transmittance at the signal wavelength. Since an idler laser did not need to be oscillated in the cavity, both the M3 and M4 mirrors were not coated with any coating for an idler laser. Traditionally, OPO for an eye-safe laser takes advantage of noncritical phase matching. This makes it possible for large effective nonlinear coefficients to be utilized and the walk-off effect can be eliminated theoretically. Even though there is not any nonlinear crystal suitable for radiating around 1730 nm, in noncritical phase matching it is also possible to attain a laser around 1730 nm by parametrical conversion. Periodically poled lithium niobate (PPLN) and periodically poled KTP (PPKTP) are promising for infrared laser generation with their high nonlinear coefficients[15,16]. However, these crystals are not adopted here because of their low damage threshold[17]. Here, the KTP crystals with a size of were cut in a special way to realize type II phase matching. The length of the OPO cavity using two nonlinear crystals is 44 mm. Placing two KTP crystals with adverse orientation can effectively reduce the walk-off effect while the effective nonlinear coefficient is also acceptable[18,19]. In order to reduce loss, both sides of the KTP crystal were AR coated at the pump, signal, and idler light. In our work we have to make the parametric resonator cavity as short as possible since the short length of the cavity leads to a low conversion threshold power density. To dissipate heat effectively, the laser rods and KTP crystal were wrapped with indium foil and tightly mounted in water-cooled heat sinks. During the experiment, the temperature of the cooling water was kept at .
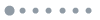
Figure 1.Schematic diagram of the experimental setup.
In this Letter, we successfully obtained the maximum power of 5.48 W at 1064 nm for the original fundamental laser for a parametric oscillator. The pulse length of the original fundamental laser was about 15 ns and the diameter of the beam reaching the OPO cavity was about 1.4 mm. These data were all measured before the laser reached the mirror M3. As the fundamental laser and signal laser will completely walk off in 20 mm, we have tried to use only one KTP crystal but failed to achieve a signal laser with a high conversion efficiency. We put the two KTP crystals with adverse orientation to reduce the walk-off effect and increase the gain length. Finally, sufficient output power is obtained. It shows that the walk-off effect cannot be ignored and adopting two KTP crystals not only decreases the walk-off effect but also increases the gain length of the nonlinear crystal. In addition, we used a dichroic mirror to separate the depleted fundamental laser and signal laser after mirror M4. The output power of the signal laser was measured after this dichroic mirror. We have adopted two kinds of M4 output mirrors at different transmittance for the signal light (, 40%). The output power of a signal laser with different transmittance couplers is shown in Fig. 2. Along with the increasing power of the original fundamental laser, we find that the power of the signal laser increases following the original fundamental laser. Here, the maximum output power of the signal laser is 1.56 W. The pulse with a pulse duration of 11.22 ns at a 500 Hz repetition rate was achieved. Fig. 2 also reflects that a lower transmittance coupler has a lower conversion threshold but lower conversion efficiency. When the power of the original fundamental laser increases a higher transmittance coupler leads to higher output power, which matches theoretical calculations. Finally, we achieve an optical conversion efficiency of 28.5% from the original fundamental laser to the signal laser. The whole system’s optical conversion efficiency here is 5.4% at the maximum output. In order to improve the power density of the original fundamental laser aiming to improve the conversion efficiency, we did not adopt any beam expander system. The peak power of the original fundamental laser reaching the crystal is about 730 kW and the peak power of the signal laser in the cavity is nearly 1112 kW. Here, if we continue raising up the original fundamental laser power, the power densities of the fundamental laser and signal laser in the cavity together will exceed the crystal damage threshold. Therefore, in order to protect the crystal from damaging, we did not continue to increase the original fundamental power.
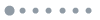
Figure 2.Output power of the original fundamental laser before and the signal laser with different output couplers versus the incident diode pump power.
Figure 3 shows the pulse waveforms of both the signal laser and the depleted fundamental laser after the output mirror at a diode pump power of 28.7 W. Here, the depleted fundamental laser and signal laser were measured simultaneously. The peak power of the signal laser is about 278 kW. A signal laser of 11.22 ns was obtained and a depleted fundamental laser pulse was 11.34 ns at the same time. There are some ripples in the pulse shape. The reason for this phenomenon is that both the depleted fundamental laser and the signal laser do not work at a single frequency, as the length of the cavity is long. Working at multiple longitudinal modes brings a beat frequency. Moreover, we have to use a high-voltage module to control the Q-switch. There are tiny fluctuations in the very beginning and the tailing of the pulse. These are caused by the high-voltage disturbance. From Fig. 3, we also can see that the signal pulse is delayed several nanoseconds after the depleted fundamental laser because the parametric conversion happens after the power density exceeds the converting threshold. We also added an original fundamental laser pulse into Fig. 3, for comparison. According to Fig. 3, the fundamental laser duration decreased from 15.7 to 11.34 ns, and we concluded that part of the original fundamental laser was converted to a signal laser according to the narrowed down pulse width.
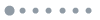
Figure 3.Pulse waveforms of the original fundamental laser, depleted fundamental laser, and signal laser.
The linewidth of the original fundamental laser at 1064.4 nm obtained was 0.06 nm, as shown in Fig. 4. The signal laser spectrum is centered on 1729.4 nm, according to Fig. 4. The spectra are all measured by an optical spectrum analyzer (AQ6370B, AQ6375, Yokogawa) with a resolution of 0.02 nm at a diode pump power of 28.7 W. The linewidth of the signal laser is about 0.1 nm, which is broad compared to the original fundamental laser because the OPO cavity cannot be absolutely stable. The original fundamental laser itself has a divergence angle so the laser beam cannot be imported absolutely parallel into the OPO. It is impossible for all the photons working at the same angle phase match, so the original fundamental laser is converted to a signal laser with a different linewidth. The fact that the waves oscillating inside the OPO cavity are diverging is also responsible for the broadened linewidth. Realizing the spectral range of the laser for the bond-select tissue imaging does not call for a narrow linewidth laser, so we do not take measures to control the linewidth.
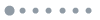
Figure 4.Spectrogram of the original fundamental laser and signal laser.
The original fundamental laser beam quality is about 1.2. Figure 5 shows a signal laser beam profile and the beam quality of the signal laser. Here we used a focal lens with a focal length of 1 m to measure the beam quality under the diode pump power of 28.7 W. The output beam quality calculated by the knife-edge scanning method is better than 1.3, similar to the value that we finally obtained of a high-beam-quality laser emitting around 1.73 μm.
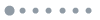
Figure 5.Beam profile and beam quality of 1.73 μm laser. Scale bar: 1 mm.
In conclusion, we describe a KTP-based OPO operating at 1729.4 nm with a good beam quality. A low-doped Nd:YAG composite crystal is used to achieve good beam quality original fundamental laser. Two KTP crystals with a special cutting angle (, ) are adopted to minimize the walk-off effect. A maximum output power of 1.56 W at 1729.4 nm is obtained under a 5.48 W pumping laser at 1064 nm with an optical conversion of 28.5% from the original fundamental laser to the signal laser. A more than 3 mJ pulse is obtained with a pulse repetition rate of 500 Hz and a pulse width of 11.22 ns. Beam quality of the output laser is better than 1.3. This laser can be used for bond-selective imaging.
References
[1] B. Hörig, F. Kühn, F. Oschütz, F. Lehmann. Int. J. Remote Sens., 22, 1413(2001).
[2] Q. Tian, Q. Tong, R. Pu, X. Guo, C. Zhao. Int. J. Remote Sens., 22, 2329(2001).
[3] R. DiFoggio, P. N. Tutunjian, H. J. Vinegar. Analytical methods and apparatus for measuring the oil content of sponge core. U. S. Patent(1989).
[4] M. Otsuka, F. Kato, Y. Matsuda, Y. Ozak. AAPS PharmSciTech, 4, 58(2003).
[5] P. Wang, H. W. Wang, M. Sturek, J. X. Cheng. J. Biophotonics, 5, 25(2012).
[6] P. Wang, T. Ma, M. N. Slipchenko, S. Liang, J. Hui, K. K. Shung, J. X. Cheng. Sci. Rep., 4, 6889(2014).
[7] S. M. Kulpa. J. Phys. Chem. Solids, 36, 1317(1975).
[8] M. E. Doroshenko, H. Jelínková, M. Němec, J. Šulc, M. Jelínek, V. K. Komar, A. S. Gerasimenko, V. M. Puzikov, V. V. Badikov. Proc. SPIE, 8599, 859921(2013).
[9] N. P. Barnes, R. Allen, L. Esterowitz, E. P. Chicklis, M. Knights, H. Jenssen. IEEE J. Quantum Electron., 22, 337(1986).
[10] Y. Wang, D. Xu, Y. Yu, W. Wen, J. Xiong, P. Wang, J. Yao. Chin. Opt. Lett., 5, 93(2007).
[11] M. Gao, C. Gao, C. K. Tang, Z. Lin, X. Zhang. Chin. Opt. Lett., 6, 127(2008).
[12] H. Li, X. Zhu, X. Ma, S. Li, C. Huang, J. Zhang, W. Chen. Chin. Opt. Lett., 12, 091401(2014).
[13] T. Sumiyoshi, Y. Otani, S. Matsumoto, H. Sekita, Y. Okagami, M. Obara. 17th Annual Meeting of the IEEE Lasers and Electro-Optics Society, 2004, LEOS 2004, 2, 807(2004).
[14] X. Peng, L. Xu, A. Asundi. IEEE J. Quantum Electron., 41, 53(2005).
[15] Y. Tang, Y. Chen, H. Jiang, W. Ji, Y. Wu, X. Chen. Chin. Opt. Lett., 11, 061901(2013).
[16] A. Chiang, Y. Y. Lin. Chin. Opt. Lett., 12, 041401(2014).
[17] B. Jiao, J. Tian, X. Zhang, Y. Song, L. Wang. Chin. Opt. Lett., 11, S21901(2013).
[18] L. R. Marshall, A. Kaz. J. Opt. Sci. Am. B, 10, 1730(1993).
[19] D. J. Armstrong, W. J. Alford, T. D. Raymond, A. V. Smith, M. S. Bowers. J. Opt. Soc. Am. B, 14, 460(1997).