
- Chinese Optics Letters
- Vol. 20, Issue 9, 091401 (2022)
Abstract
1. Introduction
Optical parametric oscillators (OPOs)[
Except for pulse energy, beam quality is another concern for distance-related applications. In fact, simple plane-parallel cavities are widely used for high energy nanosecond pulsed OPOs[
To improve the beam quality of high energy OPO systems, there are mainly two methods, one of which is the non-planar ring cavity configuration, including the rotated image singly resonant twisted rectangle (RISTRA)[
Sign up for Chinese Optics Letters TOC. Get the latest issue of Chinese Optics Letters delivered right to you!Sign up now
Another well-established method for brightness improvement is to employ unstable resonators, which are simple in structure[
In this contribution, we compared the performances of two OPO cavities, i.e., stable plane-parallel resonator and unstable resonator. A total output energy of 170 mJ was obtained in the plane-parallel cavity, where the
2. Numerical Simulation
The parametric interaction of OPOs can be described by the coupled field equations[
The pump power follows a Gaussian time distribution given by
Also, the transverse distribution of the intensity is assumed to be Gaussian, and it is given by
Using
The initial intensity of the signal wave is the vacuum fluctuations, given by Ref. [41],
The evolution of three waves can be simulated as follows, the process of which is similar to Ref. [42]. The pump pulse is injected on the crystal surface and experiences the energy conversion due to the nonlinear frequency conversion inside the nonlinear crystal. Then, the reflected waves by the output mirror become the start values of the next cavity round trip. Also, this loop will end after the pump pulse leaves the crystal.
Note that a Gaussian mirror is used in the unstable cavity OPO, whose transmission exhibits a Gaussian profile,
The calculation results are the spatial and temporal distribution of the electrical field of three waves. Subsequently, one can get the output energy of three waves using the integration, given by
The conversion efficiency of the OPO can also be calculated from the relation
Conversion efficiency is a key factor to decide the optimum OPO, and it has attracted much interest to improve the conversion efficiency recently[
Figure 1.(a) Conversion efficiency versus crystal length at 50% transmittance of the OC. (b) Conversion efficiency versus transmittance of the OC at the crystal length of 33 mm.
The efficiency of the plane-parallel cavity is approximately twice that of the unstable cavity, mainly due to the fact that the output coupler (OC) of the plane-parallel cavity is high reflection (HR) coated at 1.064 µm, whereas the OC of the unstable cavity is not. The influence of the mirror transmittance on the conversion efficiency was also analyzed, as shown in Fig. 1(b). Based on our simulations, the optimum value of the transmittance was predicted to be around 45% for our experiment. The numerical model is beneficial to optimize OPO parameters.
3. Experimental Setup
The experimental setup of the high energy KTA OPO system is depicted in Fig. 2. The system was composed of 1064 nm
Figure 2.Schematic diagram of the experimental setup of the KTA-OPO system. HR, high reflection; 90°QR, 90° quartz rotator; BP, beam polarizer; GRM1, Gaussian reflectivity mirror for 1.06 µm; ISO, isolator; M1, mirror 1; GRM2, Gaussian reflectivity mirror for 1.5 µm; M2, 45° beam splitter.
In the Nd:YAG MOPA system, the master oscillator was designed to operate at a PRF of 100 Hz
According to the simulation results, an
4. Results and Discussion
4.1. The 1064 nm pump source
The output energy of the Nd:YAG oscillator, first-stage amplifier, and second-stage amplifier is shown in Fig. 3. The maximum output pulse energy of the pump source was about 480 mJ. The typical pulse shape at the maximum output energy of the Nd:YAG MOPA system is shown in Fig. 3(a), and the pulse duration of the final output laser was about 18 ns. As shown in Fig. 3(b),
Figure 3.(a) Output energy change with the pump current. Inset shows the temporal pulse profile at maximum output power. (b) Beam quality of the energy at 480 mJ. Inset shows the beam profile.
4.2. The KTA-OPO
A beam expander system was applied to match the pump beam diameter with the crystal aperture. The diameter of the pump laser injected into the KTA-OPO was 8 mm. The crystal was wrapped with indium foil and mounted in a crystal holder. To compare the output characteristics of unstable and stable cavities, both were demonstrated with the same cavity length of 90 mm. The corresponding pulse energies of the OPO versus the pump energy are shown in Fig. 4(a). When the maximum incident pump energy was 480 mJ, the total output energy of the unstable resonator with GRM was 101 mJ, of which the 1.53 µm signal light was 75 mJ, and the 3.47 µm idler light was 26 mJ. The highest total output energy of the plane-parallel cavity was 170 mJ with the pump energy of 480 mJ. The discrepancy of nearly 70 mJ resulted from the different coating characteristics of the OCs. For the plane-parallel cavity, the OC was HR coated at 1.064 µm, enabling double-pass pumping for higher output. While limited by the coating technology, the OC (GRM2) of the unstable cavity was without HR coating for 1.064 µm, and single-pass pumping resulted in lower output energy. To date, this is the highest output level of the OPO with an unstable cavity at 100 Hz PRF. Moreover, the dependence of the output energies on the cavity length is shown in Fig. 4(b) under the stable and unstable operation. As the length of the cavity increased, the output energy of both unstable and stable cavities decreases significantly.
Figure 4.OPO output pulse energies (sum of signal and idler) for unstable and stable resonators. (a) Output energies versus the incident pump energy at the cavity length of 90 mm. (b) Output energies under different cavity lengths at the pump energy of 480 mJ.
To verify that the unstable cavity could improve the beam quality, we obtained the beam profile from a near-infrared CCD (SP620U-MIR, Ophir) and MIR (WinCamD-IR-BB, DataRay) camera. Figure 5 shows the measured beam profiles of the signal and idler, and it was found that both the signal and idler of an unstable cavity had better spatial intensity distribution than those from a stable cavity. At the same pump energy of 480 mJ, the
M2 Factors | Energy (mJ) | |||
---|---|---|---|---|
Stable | Unstable | Stable | Unstable | |
Signal | 39.8/38.4 | 9.8/9.9 | 128 | 75 |
Idler | 32.1/31.4 | 11.2/11.5 | 48 | 26 |
Table 1. Beam Quality Values and Output Energy Achieved with the Described Resonators
Figure 5.Beam quality of the signal and idler at the pump energy of 480 mJ. Inset shows the beam profile. (a) Signal of the OPO based on the plane-parallel cavity; (b) idler of the OPO based on the plane-parallel cavity; (c) signal of the OPO based on the unstable cavity with GRM; (d) idler of the OPO based on the unstable cavity with GRM.
A figure of merit that could be used to compare these two works is the brightness. The general definition of a pulsed laser source brightness is given by
Although the beam quality has been greatly improved by using a non-confocal unstable cavity with GRM, the results still need to be further improved. As reported in Ref. [30], the confocal unstable resonator has been shown to be useful for generating beams close to the diffraction limit in the OPO. To realize a confocal unstable resonator with the GRM in hand, the cavity length was increased to satisfy the confocal condition, which resulted in a significant decrease in the OPO efficiency. Building a compact confocal resonator, GRM with a short radius of curvature was required. However, it was difficult for GRMs with short radii of curvature to control damage in a high energy cavity. In future experiments, we will focus on how to use the confocal unstable cavity OPO to obtain high energy laser output with ideal beam quality. We will also improve the beam quality of the 1064 nm pump source to increase the brightness of the OPO’s output lasers.
The corresponding output spectral characteristics were measured at a maximum pulse energy of 101 mJ for the unstable cavity with GRM. As shown in Fig. 6, the bandwidth (FWHM) of the signal spectrum was approximately 0.25 nm with a central wavelength of 1535 nm. Based on the phase matching conditions, the idler laser wavelength can be calculated to be 3467 nm.
Figure 6.Spectrum of the signal light.
In addition, an indium gallium arsenide detector, an MIR detector (MIP-10-100M-F-M4, Vigo), and an oscilloscope (Wavesurfer 3034, LeCroy) were used to observe and record the pulse shape of the OPO. The results are shown in Figs. 7(a) and 7(b). At the maximum output energy, the pulse widths of the signal and idler were 16.2 ns and 15.4 ns, respectively. Figure 7(c) shows the simulation of the temporal profile of the depleted pump, signal, and idler pulses. It indicated that the signal and idler pulses build up synchronously when the pump pulse starts the conversion, and the pulse widths were estimated to be 15.6 ns and 15.8 ns for the signal and idler pulses, respectively. In general, these characteristics were basically consistent with the experimental results.
Figure 7.Typical pulse shapes of OPO based on the unstable cavity with GRM at the output energy of 101 mJ. (a) Temporal profile of the signal in the experiment; (b) temporal profile of the idler in the experiment; (c) the simulation of the temporal profile.
5. Conclusion
In summary, the brightness can be greatly improved by using a GRM and an unstable resonator as compared to the standard plane-parallel resonator. A high beam quality, high energy, unstable cavity KTA-OPO with a PRF of 100 Hz was achieved. The total output energy was about 101 mJ. The beam quality factors of the signal beam at the maximum output energy were about
References
[1] H. Kong, J. T. Bian, J. Y. Yao, Q. Ye, X. Q. Sun. Temperature tuning of BaGa4Se7 optical parametric oscillator. Chin. Opt. Lett., 19, 021901(2021).
[2] S. Q. Zha, Y. J. Chen, B. X. Li, Y. F. Lin, W. B. Liao, Y. Q. Zou, C. H. Huang, Z. L. Lin, G. Zhang. High-repetition-rate 1.5 µm passively Q-switched Er:Yb:YAl3(BO3)4 microchip laser. Chin. Opt. Lett., 19, 071402(2021).
[3] Y. H. Zhu, Z. J. Zheng, X. G. Ge, G. G. Du, S. C. Ruan, C. Y. Guo, P. G. Yan, P. Hua, L. Z. Xia, Q. T. Lü. High-power, ultra-broadband supercontinuum source based upon 1/1.5 µm dual-band pumping. Chin. Opt. Lett., 19, 041403(2021).
[4] D. H. Titterton. A review of the development of optical countermeasures. Proc. SPIE, 5615, 1(2004).
[5] H. H. P. Th. Bekman, J. C. van den Heuvel, F. J. M. van Putten, R. Schleijpen. Development of a mid-infrared laser for study of infrared counter-measures techniques. Proc. SPIE, 5615, 27(2004).
[6] G. A. Rines, D. M. Rines, P. F. Moulton. Efficient, high-energy, KTP optical parametric oscillators pumped with 1 micron Nd-lasers. Advanced Solid-State Lasers, 461(1994).
[7] Y. L. Ju, B. Q. Yao, S. Qi. High power 1.57-µm OPO pumped by MOPA with SBS. Chin. Opt. Lett., 3, 358(2005).
[8] F. F. Wang, J. T. Li, X. H. Sun, B. Z. Yan, H. K. Nie, X. Li, K. J. Yang, B. T. Zhang, J. L. He. High-power and high-efficiency 4.3 µm ZGP-OPO. Chin. Opt. Lett., 20, 011403(2022).
[9] H. Ishizuki, T. Taira. High energy quasi-phase matched optical parametric oscillation using Mg-doped congruent LiTaO3 crystal. Opt. Express, 18, 253(2010).
[10] G. Y. Liu, S. Y. Mi, K. Yang, D. S. Wei, J. H. Li, B. Q. Yao, C. Yang, T. Y. Dai, X. M. Duan, L. X. Tian, Y. L. Ju. 161 W middle infrared ZnGeP2 MOPA system pumped by 300 W-class Ho:YAG MOPA system. Opt. Lett., 46, 82(2021).
[11] J. D. Bierlein, H. Vanherzeele, A. A. Ballman. Linear and nonlinear optical properties of flux-grown KTiOAsO4. Appl. Phys. Lett., 54, 783(1989).
[12] S. Cussat-Blanc, A. Ivanov, D. Lupinski, E. Freysz. KTiOPO4, KTiOAsO4, and KNbO3 crystals for mid-infrared femtosecond optical parametric amplifiers: analysis and comparison. Appl. Phys. B, 70, 247(2000).
[13] Q. Liu, Z. L. Zhang, J. H. Liu. 100 Hz high energy KTiOAsO4 optical parametric oscillator. Infrared Phys. Technol., 61, 287(2013).
[14] Q. B. Sun, H. J. Liu, N. Huang, C. Ruan, S. L. Zhu, W. Zhao. High energy and high efficiency 3.4 µm extra cavity KTA optical parametric oscillator. Laser Phys. Lett., 8, 16(2010).
[15] Q. Liu, J. H. Liu, Z. L. Zhang, M. L. Gong. A high energy 3.75 µm KTA optical parametric oscillator at a critical angle. Laser Phys. Lett., 10, 075407(2013).
[16] J. C. McCarthy, R. C. Day, E. Chicklis. Novel, efficient, high brightness KTP optical parametric oscillator amplifier in single beamline. Advanced Solid-State Lasers, 656(2001).
[17] J. Liu, Q. Liu, L. Huang, M Gong. High energy eye-safe and mid-infrared optical parametric oscillator. Laser Phys. Lett., 7, 853(2010).
[18] J. Meng, Z. H. Cong, Z. G. Zhao, S. Wang, Y. X. Qi, X. Y. Zhang, Z. J. Liu. 100 Hz high-energy KTA dual-wavelength optical parametric oscillator. Chin. J. Lasers, 48, 1201009(2021).
[19] A. V. Smith, D. J. Armstrong. Nanosecond optical parametric oscillator with 90° image rotation: design and performance. J. Opt. Soc. Am. B, 19, 1801(2002).
[20] S. Bigotta, G. Stöppler, J. Schöner, M. Schellhorn, M. Eichhorn. Novel non-planar ring cavity for enhanced beam quality in high-pulse-energy optical parametric oscillators. Opt. Mater. Express, 4, 411(2014).
[21] M. A. Medina, M. Piotrowski, M. Schellhorn, F. R. Wagner, A. Berrou, A. Hildenbrand-Dhollande. Beam quality and efficiency of ns-pulsed high-power mid-IR ZGP OPOs compared in linear and non-planar ring resonators. Opt. Express, 29, 21727(2021).
[22] D. J. Armstrong, A. V. Smith. 150-mJ 1550-nm KTA OPO with good beam quality and high efficiency. Proc. SPIE, 5337, 71(2004).
[23] A. V. Smith, M. S. Bowers. Image-rotating cavity designs for improved beam quality in nanosecond optical parametric oscillators. J. Opt. Soc. Am. B, 18, 706(2001).
[24] R. F. Wu, K. S. Lai, H. F. Wong, W. J. Xie, Y. L. Lim, E. Lau. Multiwatt mid-IR output from a Nd:YALO laser pumped intracavity KTA OPO. Opt. Express, 8, 694(2001).
[25] S. Pearl, Y. Ehrlich, S. Fastig. Optical parametric oscillator with unstable resonators. Proc. SPIE, 4972, 58(2003).
[26] E. V. Raevsky, V. L. Pavlovitch, V. A. Konovalov. Efficient eye-safe intracavity KTP optical parametric oscillator. Proc. SPIE, 4630, 75(2002).
[27] W. A. Neuman, S. P. Velsko. Effect of cavity design on optical parametric oscillator performance. Advanced Solid-State Lasers, 179(1996).
[28] S. S. Zou, M. L. Gong, Q. Liu, G. Chen. Low threshold characteristic of pulsed confocal unstable optical parametric oscillators with Gaussian reflectivity mirrors. Opt. Express, 13, 776(2005).
[29] M. K. Brown, M. S. Bowers. High energy, near diffraction limited output from optical parametric oscillators using unstable resonators. Proc. SPIE, 2986, 113(1997).
[30] B. C. Johnson, V. J. Newell, J. B. Clark, E. S. Mcphee. Narrow-bandwidth low-divergence optical parametric oscillator for nonlinear frequency-conversion applications. J. Opt. Soc. Am. B, 12, 2122(1995).
[31] J. N. Farmer, M. S. Bowers, W. S. Schaprf. High brightness eye safe optical parametric oscillator using confocal unstable resonators. Advanced Solid-State Lasers, 567(1999).
[32] W. Scharpf, G. Ferguson, B. Boczar, M. S. Bowers, M. K. Brown. An eye safe KTA OPO with an unstable resonator. Advanced Solid-State Lasers, 189(1998).
[33] G. Hansson, H. Karlsson, F. Laurell. Unstable resonator optical parametric oscillator based on quasi-phase-matched RbTiOAsO4. Appl. Opt., 40, 5446(2001).
[34] A. I. Vodchits, V. I. Dashkevich, N. S. Kazak, V. K. Pavlenko, V. I. Pokryshkin, I. P. Petrovich, V. V. Rukhovets, A. S. Kraskovskii, V. A. Orlovich. Eye-safe radiation source based on an optical parametric oscillator. J. Appl. Spectrosc., 73, 285(2006).
[35] M. Morin. Graded reflectivity mirror unstable laser resonators. Opt. Quantum Electron, 29, 819(1997).
[36] M. Morin, M. Poirier. Graded reflectivity mirror unstable laser resonator design. Proc. SPIE, 3267, 52(1998).
[37] K. J. Snell, N. Mccarthy, M. Piché. Single transverse mode oscillation from an unstable resonator Nd:YAG laser using a variable reflectivity mirror. Opt. Commun., 65, 377(1988).
[38] S. Chandra, T. H. Allik, J. A. Hutchinson, M. S. Bowers. Improved OPO brightness with a GRM non-confocal unstable resonator. Advanced Solid-State Lasers, 177(1996).
[39] S. Zou, M. Gong, P. Yan, G. Chen, Q. Liu. Buildup time of pulsed confocal unstable optical parametric oscillator. Advanced Solid-State Photonics (TOPS), 391(2005).
[40] A. V. Smith. Crystal Nonlinear Optics: With SNLO Examples(2018).
[41] P. B. Phua, R. F. Wu, T. C. Chong. Nanosecond MIR AgGaS2 OPO and its numerical modelling. Advanced Solid State Lasers, FC14(1998).
[42] A. V. Smith, W. J. Alford, T. D. Raymond, M. S. Bowers. Comparison of a numerical model with measured performance of a seeded, nanosecond KTP optical parametric oscillator. J. Opt. Soc. Am. B, 12, 2253(1995).
[43] Z. Sacks, O. Gayer, E. Tal, A. Arie. Improving the efficiency of an optical parametric oscillator by tailoring the pump pulse shape. Opt. Express, 18, 12669(2010).
[44] T. Kawasaki, V. Yahia, T. Taira. 100 Hz operation in 10 PW/sr·cm2 class Nd:YAG Micro-MOPA. Opt. Express, 27, 19555(2019).
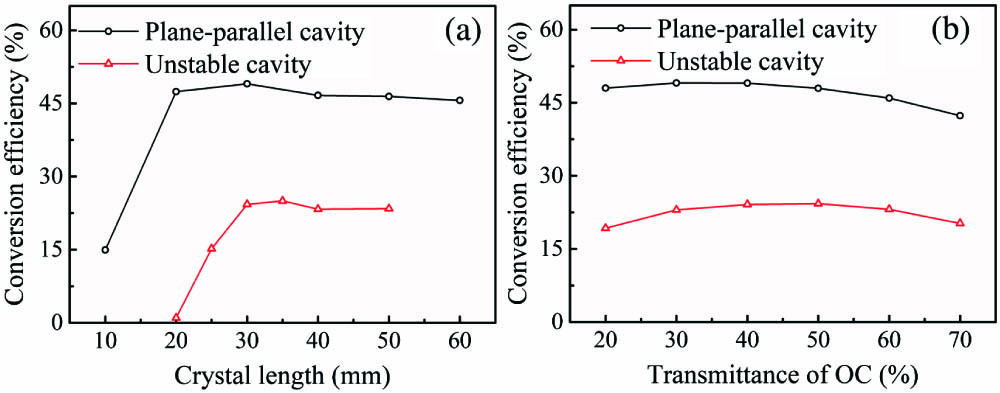
Set citation alerts for the article
Please enter your email address