The polarization beam splitter is a key component for polarization manipulation in photonic integrated circuits, but it is challenging to design for low-refractive-index optical materials, due to the low birefringence of the waveguides. We propose what we believe is a novel compact vertical-dual-slot waveguide-based coupling scheme for silicon carbide, enabling efficient low-birefringence polarization splitting by extensively modulating the transverse-magnetic mode distribution. We numerically and experimentally demonstrate the device in the 4H-silicon-carbide-on-insulator integrated platform, with a small footprint of

- Photonics Research
- Vol. 10, Issue 1, A8 (2022)
Abstract
1. INTRODUCTION
Silicon carbide (SiC) has attracted great interest in both classical and quantum integrated photonics during the past decade, thanks to its wide bandgap, excellent second- and third-order nonlinear properties, and a variety of intrinsic color centers [1,2]. These characteristics have been employed to achieve miscellaneous applications, including optical parametric oscillation, frequency comb generation, electro-optic modulation, and single-photon sources, making possible monolithic SiC quantum integrated circuits [3–8]. Among more than 200 crystalline SiC polytypes, 4H-SiC is one of the most widely used polytypes for integrated photonics, due to its high crystal quality and low optical loss [5]. As a uniaxial crystal, 4H-SiC exhibits orientation-dependent and polarization-dependent optical properties, including linear refractive index and nonlinear refractive index. It means that the transverse-electric (TE) and transverse-magnetic (TM) polarized light, propagating along the 4H-SiC waveguides, typically has different properties, such as polarization-dependent loss, polarization-dependent dispersion, and polarization-dependent nonlinear strength [9]. Thus, polarization control and management in the 4H-SiC integrated platform are highly important, and are worthy exploring.
The polarization beam splitter (PBS), a key component for polarization manipulation, enables two orthogonal mode separation and provides polarization diversity in photonic integrated circuits, which can also be applied for polarization division multiplexing and polarization entanglement quantum systems [10,11]. Many different types of PBSs have been studied in silicon-on-insulator integrated platforms (for example, directional couplers, multimode interferometers, gratings, and plasmonic waveguides) [12–17]. Although silicon (
Several methods have been proposed and demonstrated in
Sign up for Photonics Research TOC. Get the latest issue of Photonics Research delivered right to you!Sign up now
In this work, we design a novel vertical-dual-slot waveguide (VDSW) based PBS for low-birefringence waveguides. Vertical-dual-slot waveguides have been used for group velocity dispersion tailoring and efficient electro-optic modulation by enhancing the optical and electric field overlap [26,27]. Here, we use this structure to adjust the mode distribution for polarization splitting. It is found that the TM mode is particularly sensitive to the VDSW with regard to mode distribution. By adjusting the TM mode distribution, the coupling strength between the two waveguides is strongly modulated. The simple structure is also compatible with the standard single-step lithography fabrication process. We also experimentally demonstrate the PBS in the 4H-SiC-on-insulator (SiCOI) integrated platform. The device exhibits low insertion loss of
2. DESIGN AND SIMULATION
Figure 1 shows the schematic of the proposed VDSW-based PBS, and the inset shows the cross section of the coupling region of the PBS, which consists of two cores, four air slots, and three strips. The central core is a single-mode 4H-SiC waveguide and has a dimension of
Figure 1.Schematic of the proposed PBS with VDSWs.
We design and simulate the PBS using Lumerical MODE and FDTD software packages. The energy density distribution of the TE and TM modes in the 4H-SiC SMWs and the VDSWs is shown in Fig. 2. It is seen that both TE and TM modes are well confined in the SMWs and have a similar effective refractive index of 1.90 and 1.78, respectively. The effective mode area of the TE and TM modes is
Figure 2.Energy density profiles of TE and TM modes in the 4H-SiC SMWs and the VDSWs. In the simulation, the SMW has a dimension of
We optimize three parameters in the VDSW-based PBS, including the side strip width, the slot width, and the coupling length. The minimal side strip width and the slot width are limited by the minimal feature size of the fabrication process, which is about 100 nm. The coupling length of the TM mode is estimated to be 17.3 μm, according to the coupling theory of the directional couplers, given by
Figure 3.Simulated results of the transmission efficiency as a function of (a) the coupling length with a side strip width of 200 nm and a slot width of 100 nm, (b) the side strip width with a slot width of 100 nm and a coupling length of 15 μm, and (c) the slot width with a side strip width of 200 nm and a coupling length of 15 μm.
Taking comprehensive consideration of high transmission efficiency, high polarization extinction ratio, low cross talk, and fabrication feasibility, we select the side strip width, the slot width, and the coupling length of 200 nm, 100 nm, and 15 μm, respectively, as the optimized parameters of the VDSW-based PBS in the 400 nm thick 4H-SiC integrated platform. The simulated TE- and TM-polarized light propagation at 1550 nm in the optimized PBS is shown in Fig. 4. As can be seen, the TE-polarized light is directly guided in the upper waveguide, while the TM-polarized light is coupled into the adjacent waveguide, indicating that the proposed structure works effectively. The transmission efficiency and the polarization extinction ratio versus the wavelength are then simulated and plotted in Fig. 5. Within the wavelength range between 1510 and 1580 nm, both TE- and TM-polarized light beams have a high transmission efficiency of
Figure 4.(a) TE- and (b) TM-polarized light propagation along the optimized PBS.
Figure 5.Simulated results of (a) transmission efficiency and (b) polarization extinction ratio as a function of wavelength.
3. FABRICATION
After optimizing the PBS parameters, we fabricate the device on the 4H-SiCOI chip. We fabricate the 4H-SiCOI chip through the ion-cut method, shown in Fig. 6(a).
Figure 6.(a) Process flow of the 4H-SiCOI stack fabrication; (b) process flow of the 4H-SiC photonic device fabrication.
First, a high-purity semi-insulating 4H-SiC wafer is implanted with dihydrogen cations, which is on-axis cut with the extraordinary optical axis parallel to the
Figure 7.(a) SEM image of the 4H-SiCOI stack; (b) AFM image of the 4H-SiCOI surface topography within an area of
After the 4H-SiCOI chip preparation, we fabricate the device on the chip, shown in Fig. 6(b). First, the pattern is defined on the positive electron-beam (e-beam) resist, AR-P 6200.09, through the e-beam writer (JEOL JBX-9500FSZ). Second, the pattern is transferred to an aluminum metal mask through the e-beam evaporation and a lift-off process. Finally, the pattern is transferred to the 4H-SiC thin film by inductively coupled plasmon reactive ion etching. The SEM image of the fabricated device is shown in Fig. 8(a). At every input and the output port of the beam splitters, polarization-insensitive grating couplers are connected for the device performance characterization [9]. A zoom-in SEM image of the VDSW-based PBS is shown in Fig. 8(b).
Figure 8.(a) SEM image of the fabricated testing device; (b) zoom-in SEM image of the fabricated VDSW-based PBS.
4. CHARACTERIZATION
We build the measurement setup, shown in Fig. 9, to test the device. A tunable continuous-wave laser source (ANDO AQ4321D) launches light into a polarization controller to adjust the polarization to quasi-TE or quasi-TM mode. The polarized light is coupled in and out of the device with a pair of cleaved fibers mounted on the fiber holders. The output light is detected by an optical spectrum analyzer (ANDO AQ6317B), which allows synchronized sweep with the tunable laser source to measure the transmission spectrum.
Figure 9.Measurement setup schematic. CW, tunable continuous-wave laser; PC, polarization controller; OSA, optical spectrum analyzer.
The normalized transmission efficiency in the VDSW-based PBS of the TE- and TM-polarized light coupling out of Port A and Port B is plotted in Fig. 10(a). The normalized transmission has already excluded the grating transmission for each polarization, and only shows the PBS performance. As can be seen, the transmittance of the TE- and TM-polarized light in Port A and Port B, respectively, is very high and flat, within the measured wavelength range between 1520 and 1620 nm. The transmission efficiency of TE- and TM-polarized light is
Figure 10.Measured results of (a) normalized transmission efficiency and (b) polarization extinction ratio versus wavelength.
5. CONCLUSION
In this paper, a novel VDSW-based coupling scheme is proposed for low-birefringence polarization beam splitting and is experimentally demonstrated in the 4H-SiCOI integrated platform. The PBS is very small, with a footprint of
References
[1] D. M. Lukin, C. Dory, M. A. Guidry, K. Y. Yang, S. D. Mishra, R. Trivedi, M. Radulaski, S. Sun, D. Vercruysse, G. H. Ahn, J. Vučković. 4H-silicon-carbide-on-insulator for integrated quantum and nonlinear photonics. Nat. Photonics, 14, 330-334(2020).
[2] S. Castelletto, A. Boretti. Silicon carbide color centers for quantum applications. J. Phys. Photon., 2, 022001(2020).
[3] X. Shi, W. Fan, A. K. Hansen, M. Chi, A. Yi, X. Ou, K. Rottwitt, H. Ou. Thermal behaviors and optical parametric oscillation in 4H-silicon carbide integrated platforms. Adv. Photon. Res., 2, 2100068(2021).
[4] C. Wang, Z. Fang, A. Yi, B. Yang, Z. Wang, L. Zhou, C. Shen, Y. Zhu, Y. Zhou, R. Bao, Z. Li, Y. Chen, K. Huang, J. Zhang, Y. Cheng, X. Ou. High-
[5] M. A. Guidry, K. Y. Yang, D. M. Lukin, A. Markosyan, J. Yang, M. M. Fejer, J. Vučković. Optical parametric oscillation in silicon carbide nanophotonics. Optica, 7, 1139-1142(2020).
[6] M. A. Guidry, D. M. Lukin, K. Y. Yang, R. Trivedi, J. Vučković. Quantum optics of soliton microcombs(2021).
[7] T. Fan, X. Wu, S. R. Vangapandu, A. H. Hosseinnia, A. A. Eftekhar, A. Adibi. Racetrack microresonator based electro-optic phase shifters on a 3C silicon-carbide-on-insulator platform. Opt. Lett., 46, 2135-2138(2021).
[8] S. Castelletto, B. C. Johnson, A. Boretti. Quantum effects in silicon carbide hold promise for novel integrated devices and sensors. Adv. Opt. Mater., 1, 609-625(2013).
[9] X. Shi, W. Fan, Y. Lu, A. K. Hansen, M. Chi, A. Yi, X. Ou, K. Rottwitt, H. Ou. Polarization and spatial mode dependent four-wave mixing in a 4H-silicon carbide microring resonator. APL Photon., 6, 076106(2021).
[10] Y. Tan, H. Wu, D. Dai. Silicon-based hybrid (de)multiplexer for wavelength-/polarization-division-multiplexing. J. Lightwave Technol., 36, 2051-2058(2018).
[11] E. Meyer-Scott, N. Prasannan, C. Eigner, V. Quiring, J. M. Donohue, S. Barkhofen, C. Silberhorn. High-performance source of spectrally pure, polarization entangled photon pairs based on hybrid integrated-bulk optics. Opt. Express, 26, 32475-32490(2018).
[12] J. Wang, D. Liang, Y. Tang, D. Dai, J. E. Bowers. Realization of an ultra-short silicon polarization beam splitter with an asymmetrical bent directional coupler. Opt. Lett., 38, 4-6(2013).
[13] H. Zafar, M. F. Pereira, K. L. Kennedy, D. H. Anjum. Fabrication-tolerant and CMOS-compatible polarization splitter and rotator based on a compact bent-tapered directional coupler. AIP Adv., 10, 125214(2020).
[14] C. Errando-Herranz, S. Das, K. B. Gylfason. Suspended polarization beam splitter on silicon-on-insulator. Opt. Express, 26, 2675-2681(2018).
[15] D. Dai, Z. Wang, J. Peters, J. E. Bowers. Compact polarization beam splitter using an asymmetrical Mach–Zehnder interferometer based on silicon-on-insulator waveguides. IEEE Photon. Technol. Lett., 24, 673-675(2012).
[16] C. Li, M. Zhang, J. E. Bowers, D. Dai. Ultra-broadband polarization beam splitter with silicon subwavelength-grating waveguides. Opt. Lett., 45, 2259-2262(2020).
[17] J. Chee, S. Zhu, G. Lo. CMOS compatible polarization splitter using hybrid plasmonic waveguide. Opt. Express, 20, 25345-25355(2012).
[18] S. Gao, Y. Wang, K. Wang, E. Skafidas. Low-loss and broadband 2 ×2 polarization beam splitter based on silicon nitride platform. IEEE Photon. Technol. Lett., 28, 1936-1939(2016).
[19] R. Kudalippalliyalil, T. E. Murphy, K. E. Grutter. Low-loss and ultra-broadband silicon nitride angled MMI polarization splitter/combiner. Opt. Express, 28, 34111-34122(2020).
[20] H. Xu, D. Dai, L. Liu, Y. Shi. Proposal for an ultra-broadband polarization beam splitter using an anisotropy-engineered Mach-Zehnder interferometer on the
[21] J. Zhan, J. Brock, S. Veilleux, M. Dagenais. Silicon nitride polarization beam splitter based on polarization-independent MMIS and apodized Bragg gratings. Opt. Express, 29, 14476-14485(2021).
[22] C. Deng, M. Lu, Y. Sun, L. Huang, D. Wang, G. Hu, R. Zhang, B. Yun, Y. Cui. Broadband and compact polarization beam splitter in LNOI hetero-anisotropic metamaterials. Opt. Express, 29, 11627-11634(2021).
[23] L. Zhang, X. Fu, L. Yang. Compact, broadband and low-loss polarization beam splitter on lithium-niobate-on-insulator using a silicon nanowire assisted waveguide. IEEE Photon. J., 12, 6601906(2020).
[24] J. Feng, R. Akimoto. A three-dimensional silicon nitride polarizing beam splitter. IEEE Photon. Technol. Lett., 26, 706-709(2014).
[25] B. Bhandari, C.-S. Im, O. R. Sapkota, S.-S. Lee. Highly efficient broadband silicon nitride polarization beam splitter incorporating serially cascaded asymmetric directional couplers. Opt. Lett., 45, 5974-5977(2020).
[26] S. Shi, D. W. Prather. Ultrabroadband electro-optic modulator based on hybrid silicon-polymer dual vertical slot waveguide. Adv. Optoelectron., 2011, 714895(2011).
[27] K. Guo, L. Lin, J. B. Christensen, E. N. Christensen, X. Shi, Y. Ding, K. Rottwitt, H. Ou. Broadband wavelength conversion in a silicon vertical-dual-slot waveguide. Opt. Express, 25, 32964-32971(2017).
[28] B. E. Little, W.-P. Huang. Coupled-mode theory for optical waveguides. Prog. Electromagn. Res., 10, 217-270(1995).
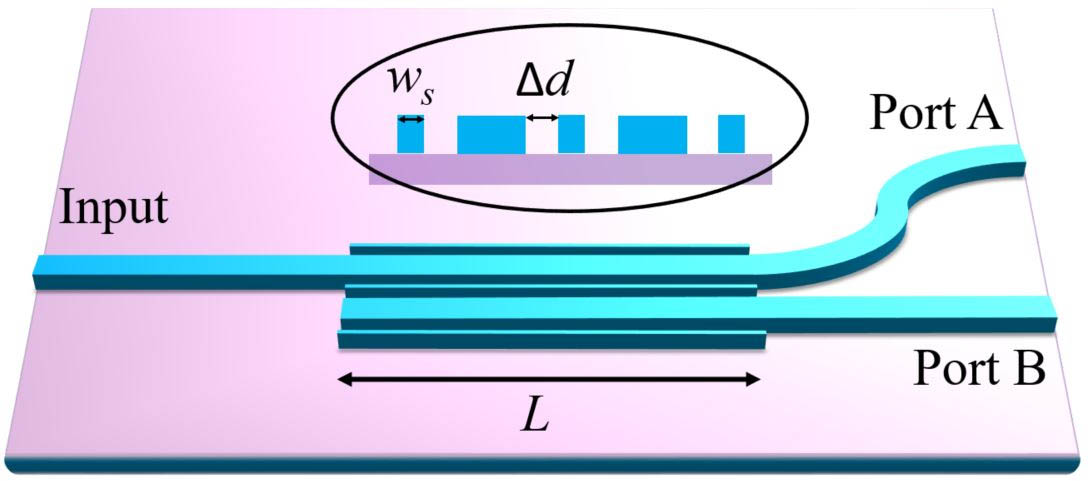
Set citation alerts for the article
Please enter your email address