N. V. Kryzhanovskaya1、*, E. I. Moiseev1, F. I. Zubov1, A. M. Mozharov1, M. V. Maximov1, N. A. Kalyuzhnyy2, S. A. Mintairov2, M. M. Kulagina2, S. A. Blokhin2, K. E. Kudryavtsev3, A. N. Yablonskiy3, S. V. Morozov3, Yu. Berdnikov4, S. Rouvimov5, and A. E. Zhukov1
Author Affiliations
1St. Petersburg Academic University, Khlopina 8/3, St. Petersburg, 194021, Russia2Ioffe Institute of RAS, Politehnicheskaya 26, St. Petersburg, 194021, Russia3Institute for Physics of Microstuctures of RAS, Nizhny Novgorod, 603950, Russia4ITMO University, Kronverkskiy prospekt 49, St. Petersburg, 197101, Russia5University of Notre Dame, Notre Dame, Indiana 46556, USAshow less
Abstract
GaAs-based microdisk lasers with an active region representing a dense array of indium-rich islands (InGaAs quantum well-dots) were studied using direct small-signal modulation. We demonstrate that using dense arrays of InGaAs quantum well-dots enables uncooled high-frequency applications with a GHz-range bandwidth for microdisk lasers. A maximum 3?dB modulation frequency of 5.9?GHz was found in the microdisk with a radius of 13.5?μm operating without a heatsink for cooling. A modulation current efficiency factor of was estimated.1. INTRODUCTION
Whispering-gallery mode (WGM) lasers, such as microring and microdisk lasers, with small footprints and in-plane emissions, have been widely investigated as efficient and compact light sources. Historically, the first WGM lasers were demonstrated with spheres in the early 1960s [1]. The first injection WGM microlasers represented microdisk resonators with InGaAs/InGaAsP quantum well active regions [2]. Since then there has been significant progress in the development of InP-based microlasers and their hybrid integration with silicon. In particular, high-speed direct modulation with a 3 dB bandwidth of 11.7 GHz and open eye diagrams at 10 Gb/s were demonstrated for an InGaAsP/InP microdisk device with a diameter of 7.5 μm bonded to silicon [3]. For AlGaInAs/InP microdisk lasers with radii of 15 and 10 μm, and bandwidths of 8.7 and 13 GHz, respectively, were realized [4]. Later, a small signal modulation response with a 3 dB bandwidth up to 20 GHz was obtained for a similar microdisk laser with a radius of 7 μm [5]. However, those InP-based microlasers are strongly temperature dependent. With the increase of temperature by only 26°C (from 14 to 40°C), the resonance frequencies decrease by about 45% (from 14.9 GHz to about 8.3 GHz). Small energy offsets and low thermal conductivity of quaternary alloys make InP-based microlasers temperature sensitive [6]. This requires a thermoelectric cooler to stabilize the device temperature, which brings additional power consumption, increases size, and limits its use in many applications.
Self-organized In(Ga)As/GaAs quantum dots (QDs) can offer much better temperature stability owing to the three-dimensional confinement of deeply localized carriers, the high-optical contrast achievable in AlGaAs/GaAs heterostructures, and their better thermal conductivity. For example, a specific thermal resistance evaluated for QD microdisk lasers with diameters ranging from 2 μm to 30 μm is approximately 2.4 times lower than that for microlasers on InP substrates [7]. QD edge-emitting lasers have been realized that operate up to 220°C [8]. Data transmission with a bit rate of 10 Gb/s was attained under fixed modulation and bias currents across a wide temperature range from 20°C to 90°C [9]. As for QD microlasers, lasing under injection pumping was first demonstrated at 5 K with InAs QDs in 2003 [10]. Eventually, lasing was achieved at room and elevated temperatures (e.g., CW lasing at 50°C with InAs/InGaAs QD microlasers [11]). CW operation of uncooled 31 μm diameter microdisk lasers up to 100°C with similar InAs/InGaAs QDs was attained [12]. Due to more than an order of magnitude reduced diffusion length compared to a quantum well [13], QDs are characterized by a reduced sensitivity to nonradiative recombination at deeply etched sidewalls [14], which is especially important for microlasers. QDs are also less sensitive to threading dislocations and other growth defects inevitable for III-V on Si growth. Owing to this fact, QD-based microlasers monolithically grown on silicon, which are capable of operating at room and elevated temperatures, have been recently reported [15,16]. Meanwhile, the reports on the dynamic characteristics of QD-based microdisk/microring lasers are practically absent. In this regard, mention should be made of recent work [17], in which QD-on-Si microring lasers of a relatively large radius of 50 μm were investigated, and a 3 dB bandwidth of 6.5 GHz was achieved.
The optical gain achievable on the QD ground state optical transition is limited due to the finite number of QDs. When the resonator diameter decreases below a certain value or the temperature rises above a certain value, a transition to the excited state lasing occurs [16,18]. Another drawback of self-organized QDs is an unusually high gain compression coefficient [19–21], which is one or two orders of magnitude greater than typical values for quantum well lasers and affects the modulation bandwidth. Recently we reported on microdisk lasers with a novel sort of nanostructures in the active region, referred to as quantum well-dots (QWDs) [22,23]. These QWDs are ultradense (several ) uniform arrays of In-rich islands inside an In-depleted residual quantum well [24]. Owing to the high density of the islands, a higher optical gain is achieved compared to that of conventional QDs, whereas the lateral carrier transport is suppressed as opposed to a quantum well. With QWDs, a high output power of 18 mW and a peak electrical-to-optical power conversion efficiency of 15% were demonstrated in a 31 μm diameter microdisk laser [25]. CW lasing was observed up to 110°C [25].
Sign up for Photonics Research TOC. Get the latest issue of Photonics Research delivered right to you!Sign up now
In the present work, we report on the dynamic characteristics of microdisk lasers on GaAs with InGaAs QWDs in the active region. We achieved a room-temperature modulation bandwidth slightly below 6 GHz in the microdisk lasers with a radius of 13.5 μm operating without external cooling. No sign of the gain compression was found.
2. MICROLASER FABRICATION AND STATIC PERFORMANCE
An epitaxial wafer was grown on an n+-GaAs(100)6° substrate by low pressure metal-organic vapor phase epitaxy with hydrogen as a carrier gas, arsine as a group V precursor, trimethylgallium, trimethylindium, and trimethyl-aluminum as group III precursors, and silane and diethylzinc as n-type and p-type dopants. An laser heterostructure comprises a 0.8 μm thick GaAs waveguide with 5 layers of QWDs formed by the deposition of 8 monolayers and separated with 40 nm thick GaAs spacers. Laser microresonators were formed by single-step photolithography and dry etching of 5.5 μm high mesas with nearly vertical sidewalls (STE ICPe68 setup). Ohmic contacts were formed with AgMn/NiAu and AuGe/Ni/Au metallizations upon the p+ GaAs cap layer and the n-doped GaAs substrate, respectively (Fig. 1). A bright-field transmission electron microscopy (TEM) image of the active region cross section is depicted in the inset of Fig. 1. The variation in contrast in layers is related to strain and changes in composition along the layer, revealing the presence of In-rich islands.
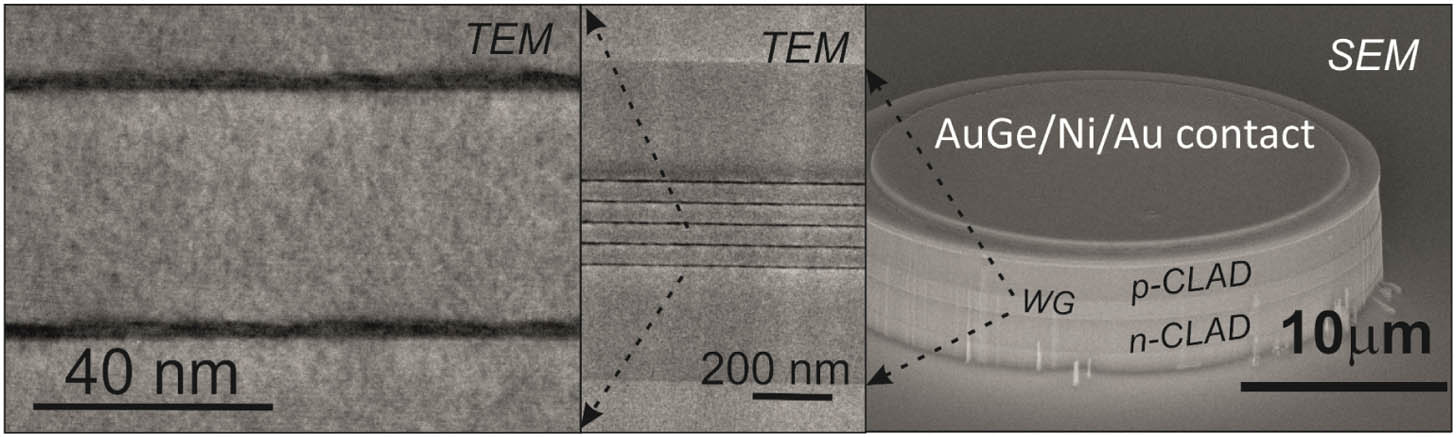
Figure 1.Scanning electron microscopy (SEM) image of microdisk laser and transmission electron microscopy (TEM) images of the active region cross section.
Microdisk planarization was done using epoxy resist SU-8. A group of 12 microlasers with a common bottom contact was soldered on a holder suitable for connection using a ground–signal–ground (GSG) radio-frequency (RF) probe. All measurements were done at room temperature without external temperature stabilization and cooling. The top contact of an individual microlaser was connected using a 20 μm thick gold wire. Microlasers with a diameter of 27 μm were selected for testing. The external efficiency of those lasers is sufficiently high to use the light emitted into free space to evaluate the dynamic characteristics.
Light–current–voltage (L-I-V) curves were measured in a continuous-wave regime. Light was collected with a Thorlabs FDG0110c germanium photodiode. Emission spectra were measured with a spectral resolution of using a Bruker IFS125HR Fourier spectrometer. Electroluminescence was excited by a stabilized DC current with a Keithley 2400 Series SourceMeter and detected with an uncooled InGaAs photodetector. Dynamic characteristics were measured in the range between 50 MHz and 20 GHz with a New Focus 1434 25 GHz photodetector and an Agilent E8364B network analyzer. The RF signal was combined with the DC bias through a high-frequency bias tee and fed to microlaser by a high-frequency GSG probe head.
In different microlasers, the lasing threshold varies from 8 to 10 mA, which corresponds to the average threshold current density of about . Emission spectra taken below and above the threshold are depicted in Fig. 2. A series of WGMs is observed with a free spectral range of that corresponds to the effective group index of . The dominant (most intense) mode appears at (1082 nm). The lasing threshold is further confirmed by the characteristic decrease of the linewidth of the dominant WGM line, as shown in the inset in Fig. 2. The mode full width at half maximum (FWHM) drops down to () at and then slightly increases. Since the FWHM broadening is correlated with a thermal red shift of the mode (slope is approximately ), this behavior can be attributed to heating of the microlaser [26].
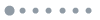
Figure 2.Emission spectra below (dotted line) and above (solid line) the lasing threshold; inset: mode linewidth versus normalized current.
Figure 3 shows a representative characteristic of the microlaser. The output power collected by the photodiode is on the order of 100 μW. The slope efficiency of about 50–100 μW/mA was estimated for different microlasers. For larger microlasers () without SU-8 planarization, we have recently reported a slope efficiency of 0.35 mW/mA [25]. At an injection current of 30 mA, the voltage is 1.9 V and the differential resistance was estimated to be 12 Ω. Figure 3 also depicts the integrated intensity of the dominant mode line extracted from the emission spectra. A characteristic kink at the lasing threshold is clearly observed.
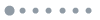
Figure 3.Integrated intensity of the dominant mode (circles) and total emitted power (triangles) as functions of current and current voltage characteristic (squares) of a microdisk laser. Arrow denotes the lasing threshold.
3. DYNAMIC PERFORMANCE
The small signal modulation responses for different bias currents are shown in Fig. 4. The 3 dB bandwidth, , is presented as a function of the injection current in Fig. 5. Closer to the lasing threshold, the experimental data can be described well as using the modulation current efficiency factor value (MCEF) of as a fitting parameter (solid line in Fig. 5). For the microdisk with a diameter of 27 μm the maximal of 5.9 GHz was achieved at a bias current of 30 mA.
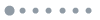
Figure 4.Experimental (symbols) and fitted (curve) small signal modulation responses at different bias currents.
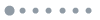
Figure 5.Experimental data at 3 dB bandwidth (solid symbols) and relaxation oscillation frequency (open symbols) against the squared root of the injection current.
The experimental modulation response curves were fitted using the following expression resulting from the rate equation theory [27], where is the relaxation oscillation frequency, is the damping rate, and is the cutoff frequency associated with parasites and/or carrier transport to the active region. The parameters extracted provide reasonable fit of the experimental data for all injection currents. Example of the fitting curve is shown in Fig. 4 for the bias that corresponds to the highest 3 dB bandwidth. The relaxation oscillation frequency is presented as a function of current in Fig. 5. Using an expression , the factor of was found by fitting the near-threshold experimental data (dashed line in Fig. 5). This is comparable to a factor of for 15 μm radius microdisk laser made of the InP-based heterostructure [28]. At higher currents, the oscillation frequency is saturated with a maximum value of about 5 GHz.
Combining the data presented in Figs. 3 and 5, we plot the squared relaxation oscillation frequency as a function of the lasing power , as shown in Fig. 6. This relationship is used to evaluate the gain compression coefficient [29], . Up to the values of the lasing power, at which the relaxation oscillation frequency is saturated due to thermal effects, there is no deviation from the linear dependence. Hence we conclude that the gain compression has a negligible impact on the dynamic characteristics of the microdisk lasers under study.
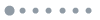
Figure 6.Squared relaxation oscillation frequency against the lasing power. Line is a guide for the eye.
The damping rate versus squared relaxation oscillation frequency is shown in Fig. 7. The linear fit by using equation gives the K-factor of 0.7 ns and the damping factor offset of 3.5 GHz. The maximal intrinsic bandwidth was then calculated using equation [30] and found to be 13 GHz, which is noticeably higher than the maximal . Thus, we conclude that the modulation bandwidth of the microlasers under study is not the K-factor limited. The value of the K-factor itself is determined by the photon lifetime in the cavity and the gain compression. If the latter is ignored, the photon lifetime can be estimated as of about 18 ps. This corresponds to the optical cavity loss of . In turn, this allows us to estimate the loss-limited linewidth of the bare cavity as 35 pm. Compared to the linewidth data presented in the inset in Fig. 2, one can conclude that the transparency current (at which the net gain of the active region is zero) is about .
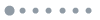
Figure 7.Experimental (symbols) and fitted (curve) damping factor versus squared relaxation oscillation frequency.
In a quantum-dot laser, the transport time is determined by the carrier capture into the active region and their relaxation to the ground-state level [29,31]. For Stranski–Krastanow QDs, these times are about a few picoseconds [32,33], and there is no reason to suppose that the heterostructures considered in the present work are characterized by a slower carrier capture/relaxation. The extracted values of the cutoff frequency are scattered around 6 GHz. The thermally limited modulation bandwidth is given by equation [27,34] and corresponds to a maximum thermal bandwidth limit of 6.8 GHz, which is very close to . Since the intrinsic resonance improves the parasitic-limited response at high frequencies, further studies are required to clarify which mechanism is predominantly responsible for the bandwidth limitation.
4. CONCLUSIONS
To conclude, we show that using dense arrays of InGaAs quantum well-dots enables uncooled high-frequency applications with a GHz-range bandwidth for microdisk lasers. To the best of our knowledge, these microlasers are the smallest microdisk/microring lasers on GaAs with the InGaAs quantum well-dot active region, for which a direct modulation has been realized. A modulation current efficiency factor of was estimated, and bias currents, at which the maximal modulation frequency is achieved, are in the range of 30 mA (for comparison, MCEF of and injected current of 86 mA were reported in Ref. [17]). Higher -factor and MCEF are expected for smaller microlaser radii (for example, a -factor increase from 1.36 to was reported for temperature-stabilized AlGaInAs/InP microdisks as their radius decreases from 15 to 7 μm [5]). No impact of the gain compression on the dynamic characteristics was found. The K-limited maximal frequency of 13 GHz was estimated from the modulation response analysis, whereas the experimentally measured 3 dB modulation frequency was 5.9 GHz. We suggest the bandwidth is limited by thermal effects or RC parasites, or a combination of those two things. Higher Q-factors of the microcavity and, as a result, lower threshold current density can bring more opportunities to QD microlasers. Very high Q-factors in excess of have been achieved in silica and some other materials (see Ref. [35] and references therein). However, Q-factors of microdisks made of III-V materials are not so high. This emphasizes the importance of further development of III-V microdisk fabrication technology. In addition, the sidewall passivation can be useful to suppress the nonradiative component of the recombination current, while coating the microdisk with materials with high thermal conductivity can help prevent overheating of the active region.
References
[1] C. G. B. Garrett, W. Kaiser, W. L. Bond. Stimulated emission into optical whispering gallery modes of spheres. Phys. Rev., 124, 1807-1809(1961).
[2] A. F. J. Levi, R. E. Slusher, S. L. McCall, T. Tanbuk-Ek, D. L. Coblentz, S. J. Perton. Room temperature operation of microdisc lasers with submilliamp threshold current. Electron. Lett., 28, 1010-1012(1992).
[3] J. Hofrichter, O. Raz, S. Keyvaninia, T. de Vries, H. J. S. Dorren, T. Morf, B. J. Offrein. High-speed direct-modulation of InP microdisk lasers. 39th European Conference and Exhibition on Optical Communication (ECOC), We.1.B.5(2013).
[4] X. M. Lv, Y. Z. Huang, L. X. Zou, H. Long, Y. Du. Optimization of direct modulation rate for circular microlasers by adjusting mode Q factor. Laser Photon. Rev., 7, 818-829(2013).
[5] L.-X. Zou, Y.-Z. Huang, B.-W. Liu, X.-M. Lv, X.-W. Ma, Y.-D. Yang, J.-L. Xiao, Y. Du. Thermal and high speed modulation characteristics for AlGaInAs/InP microdisk lasers. Opt. Express, 23, 2879-2888(2015).
[6] A. K. Sokol, R. P. Sarzala. Comparative analysis of thermal problems in GaAs- and InP-based 1.3-μm VECSELs. Opt. Appl., 43, 325-341(2013).
[7] N. V. Kryzhanovskaya, M. V. Maximov, S. A. Blokhin, M. A. Bobrov, M. M. Kulagina, S. I. Troshkov, Y. M. Zadiranov, A. A. Lipovskii, E. I. Moiseev, Y. V. Kudashova, D. A. Livshits, V. M. Ustinov, A. E. Zhukov. Microdisk injection lasers for the 1.27-μm spectral range. Semiconductors, 50, 390-393(2016).
[8] T. Kageyama, K. Nishi, M. Yamaguchi, R. Mochida, Y. Maeda, K. Takemasa, Y. Tanaka, T. Yamamoto, M. Sugawara, Y. Arakawa. Extremely high temperature (220°C) continuous-wave operation of 1300-nm-range quantum-dot lasers. Conference on Lasers and Electro-Optics Europe and 12th European Quantum Electronics Conference (CLEO/EQEC), PDA_1(2011).
[9] M. Ishida, N. Hatori, K. Otsubo, T. Yamamoto, Y. Nakata, H. Ebe, M. Sugawara, Y. Arakawa. Low-driving-current temperature-stable 10 Gbit/s operation of p-doped 1.3 μm quantum dot lasers between 20 and 90°C. Electron. Lett., 43, 219-221(2007).
[10] L. Zhang, E. Hu. Lasing from InGaAs quantum dots in an injection microdisk. Appl. Phys. Lett., 82, 319-321(2003).
[11] M. Munsch, J. Claudon, N. S. Malik, K. Gilbert, P. Grosse, J.-M. Gerard, F. Albert, F. Langer, T. Schlereth, M. M. Pieczarka, S. Hofling, M. Kamp, A. Forchel, S. Reitzenstein. Room temperature, continuous wave lasing in microcylinder and microring quantum dot laser diodes. Appl. Phys. Lett., 100, 031111(2012).
[12] N. V. Kryzhanovskaya, E. I. Moiseev, Y. V. Kudashova, F. I. Zubov, A. A. Lipovskii, M. M. Kulagina, S. I. Troshkov, Y. M. Zadiranov, D. A. Livshits, M. V. Maximov, A. E. Zhukov. Continuous-wave lasing at 100°C in 1.3 μm quantum dot microdisk diode laser. Electron. Lett., 51, 1354-1355(2015).
[13] A. Fiore, M. Rossetti, B. Alloing, C. Paranthoen, J. X. Chen, L. Geelhaar, H. Riechert. Carrier diffusion in low-dimensional semiconductors: a comparison of quantum wells, disordered quantum wells, and quantum dots. Phys. Rev. B, 70, 205311(2004).
[14] D. Ouyang, N. N. Ledentsov, D. Bimberg, A. R. Kovsh, A. E. Zhukov, S. S. Mikhrin, V. M. Ustinov. High performance narrow stripe quantum-dot lasers with etched waveguide. Semicond. Sci. Technol., 18, L53-L54(2003).
[15] Y. Wan, J. Norman, Q. Li, M. J. Kennedy, D. Liang, C. Zhang, D. Huang, Z. Zhang, A. Y. Liu, A. Torres, D. Jung, A. C. Gossard, E. L. Hu, K. M. Lau, J. E. Bowers. 1.3 μm submilliamp threshold quantum dot micro-lasers on Si. Optica, 4, 940-944(2017).
[16] N. V. Kryzhanovskaya, E. I. Moiseev, Y. S. Polubavkina, M. V. Maximov, D. V. Mokhov, I. A. Morozov, M. M. Kulagina, Y. M. Zadiranov, A. A. Lipovskii, M. Tang, M. Liao, J. Wu, S. Chen, H. Liu, A. E. Zhukov. Elevated temperature lasing from injection microdisk lasers on silicon. Laser Phys. Lett., 15, 015802(2018).
[17] Y. Wan, D. Inoue, D. Jung, J. C. Norman, C. Shang, A. C. Gossard, J. E. Bowers. Directly modulated quantum dot lasers on silicon with a milliampere threshold and high temperature stability. Photon. Res., 6, 776-781(2018).
[18] M. V. Maximov, N. V. Kryzhanovskaya, A. M. Nadtochiy, E. I. Moiseev, I. I. Shostak, A. A. Bogdanov, Z. F. Sadrieva, A. E. Zhukov, A. A. Lipovskii, D. V. Karpov, J. Laukkanen, J. Tommila. Ultrasmall microdisk and microring lasers based on InAs/InGaAs/GaAs quantum dots. Nanoscale Res. Lett., 9, 657(2014).
[19] F. Grillot, B. Dagens, J.-G. Provost, H. Su, L. F. Lester. Gain compression and above-threshold linewidth enhancement factor in 1.3-μm InAs-GaAs quantum-dot lasers. IEEE J. Quantum Electron., 44, 946-951(2008).
[20] D.-Y. Cong, A. Martinez, K. Merghem, A. Ramdane, J.-G. Provost, M. Fischer, I. Krestnikov, A. Kovsh. Temperature insensitive linewidth enhancement factor of p-type doped InAs/GaAs quantum-dot lasers emitting at 1.3 μm. Appl. Phys. Lett., 92, 191109(2008).
[21] A. E. Zhukov, M. V. Maximov, N. Y. Gordeev, A. V. Savelyev, D. A. Livshits, A. R. Kovsh. Quantum dot lasers with controllable spectral and modal characteristics. Semicond. Sci. Technol., 26, 014004(2011).
[22] S. A. Mintairov, N. A. Kalyuzhnyy, M. V. Maximov, A. M. Nadtochiy, S. Rouvimov, A. E. Zhukov. GaAs quantum well-dots solar cells with spectral response extended to 1100 nm. Electron. Lett., 51, 1602-1604(2015).
[23] S. A. Mintairov, N. A. Kalyuzhnyy, M. V. Maximov, A. M. Nadtochiy, A. E. Zhukov. InGaAs quantum well-dots based GaAs subcell with enhanced photocurrent for multijunction GaInP/GaAs/Ge solar cells. Semicond. Sci. Technol., 32, 015006(2017).
[24] S. A. Mintairov, N. A. Kalyuzhnyy, V. M. Lantratov, M. V. Maximov, A. M. Nadtochiy, S. Rouvimov, A. E. Zhukov. Hybrid InGaAs quantum well-dots nanostructures for light-emitting and photo-voltaic applications. Nanotechnology, 26, 385202(2015).
[25] E. Moiseev, N. Kryzhanovskaya, M. Maximov, F. Zubov, A. Nadtochiy, M. Kulagina, Y. Zadiranov, N. Kalyuzhnyy, S. Mintairov, A. Zhukov. Highly efficient injection microdisk lasers based on quantum well-dots. Opt. Lett., 43, 4554-4557(2018).
[26] P. Jaffrennou, J. Claudon, M. Bazin, N. S. Malik, S. Reitzenstein, L. Worschech, M. Kamp, A. Forchel, J.-M. Gerard. Whispering gallery mode lasing in high quality GaAs/AlAs pillar microcavities. Appl. Phys. Lett., 96, 071103(2010).
[27] L. A. Coldren, S. W. Corzine, M. L. Masanovic. Diode Lasers and Photonic Integrated Circuits(2012).
[28] X. M. Lv, Y. Z. Huang, Y. D. Yang, L. X. Zou, H. Long, B. W. Liu, J. L. Xiao, Y. Du. Influences of carrier diffusion and radial mode field pattern on high speed characteristics for microring lasers. Appl. Phys. Lett., 104, 161101(2014).
[29] H. Su, L. F. Lester. Dynamic properties of quantum dot distributed feedback lasers: high speed, linewidth and chirp. J. Phys. D, 38, 2112-2118(2005).
[30] E. Kapon. Semiconductor Lasers: Fundamentals(1999).
[31] P. Bhattacharya, D. Klotzkin, O. Qasaimeh, W. Zhou, S. Krishna, D. Zhu. High-speed modulation and switching characteristics of In(Ga)As-Al(Ga)As self-organized quantum-dot lasers. IEEE J. Sel. Top. Quantum Electron., 6, 426-438(2000).
[32] M. Ishida, N. Hatori, T. Akiyama, K. Otsubo, Y. Nakata, H. Ebe, M. Sugawara, Y. Arakawa. Photon lifetime dependence of modulation efficiency and K factor in 1.3 μm self-assembled InAs/GaAs quantum-dot lasers: Impact of capture time and maximum modal gain on modulation bandwidth. Appl. Phys. Lett., 85, 4145-4147(2004).
[33] A. Fiore, A. Markus. Differential gain and gain compression in quantum-dot lasers. IEEE J. Quantum Electron., 43, 287-294(2007).
[34] A. N. AL-Omari, I. K. AL-Kofahi, K. L. Lear. Fabrication, performance and parasitic parameter extraction of 850 nm high-speed vertical-cavity lasers. Semicond. Sci. Technol., 24, 095024(2009).
[35] Q. H. Song. Emerging opportunities for ultra-high Q whispering gallery mode microcavities. Sci. China: Phys. Mech. Astron., 62, 074231(2019).