
- Chinese Optics Letters
- Vol. 20, Issue 2, 021601 (2022)
Abstract
1. Introduction
Structural colors can achieve optical effects, such as interference, diffraction, and scattering of light by using the fine micro-nanostructure on the surface of organisms, showing gorgeous colors[
The basic working principle of color design is the thin-film interference effect of light. When the incident angle of light changes, the spectral properties of the thin films will also change, resulting in different colors[
In this study, we demonstrate a silver (Ag)-based simple five-layer structure in order to compress short-wave sub-peak and reduce long-wavelength absorption. Using the absorption characteristics of a-Si, silicon monoxide (SiO) and other materials realize the selective absorption of the spectrum, so that the absorption of the thin films is high at the shorter wavelengths but low at the longer wavelengths. The absorption of the proposed structure is more than 96% below 550 nm, and it gradually decreases to 8% in the 650–750 nm region. Meanwhile, we use antireflection (AR) layers to further suppress the remaining sideband reflection. We designed and produced a five-layer red structural color. This structure is based on metallic Ag, which has high reflection, low short-wavelength reflection, and high long-wavelength reflection. The average residual reflection of this structure in the 400–550 nm wavelength range is 2.35%, the average reflection of red range (650–750 nm) is 78.65%, and the peak reflection is 91%. The colorimetric purity of the red color obtained through experiment is 0.9. The thin films are simple in structure, and finally realize red structural color with low angle sensitivity, high color purity, and high brightness. This one-dimensional lamellar thin-film structure is easy to mass produce through the conventional physical vapor deposition (PVD) process. It has a wide range of applications in optical display, optical anti-counterfeit, decoration, stealth, and other fields.
Sign up for Chinese Optics Letters TOC. Get the latest issue of Chinese Optics Letters delivered right to you!Sign up now
2. Structure and Design
The focus of our design is to create an angle-insensitive red color with higher purity and higher brightness. At visible wavelengths, red belongs to the long wave, and the color in the region of the short wavelength will affect the purity of red. To solve this problem, we increase short-wavelength absorption and use AR layers. The designed five-layer structure, which can produce angle-insensitive red color in the range of 0° to 60°, is shown in Fig. 1(a). The structure has a high color purity, which is achieved by using the overlapping of high and low refractive index materials to produce steep reflection in the long-wave range and suppress sideband reflection.
Figure 1.(a) Schematic view of the proposed structure. (b) Optical constants of a-Si (180 nm) under different experimental conditions. (c) Optical constants of a-Si in the design. (d) Optical constants of SiO.
Figure 1(a) shows a schematic diagram of the proposed structure, which consists of four materials with different refractive indices alternately stacked. The bottom layer is Ag with the thickness of 100 nm. In our study, Ag is selected as the material of the bottom metal because it has the lowest material absorption loss and the highest reflectivity in all visible wavelength ranges of all metals. In the 400–800 nm band, the refractive index of Ag varies from 0.05 to 0.09, and the extinction coefficient varies from 1.93 to 5.45. The thickness of the
For the color design in the spectrum, it is necessary to compress the reflection bandwidth and eliminate the reflection sub-peak for improving color purity. Since a-Si is a kind of material with high refractive index, the reflective index difference between a-Si and air is large, which will lead to a considerable amount of reflection in the 400–600 nm wavelength range. So, it affects the purity of the red color. The top two layers of the structure, SiO and
This is the equation of a circle whose center coordinate is
Figure 2.Optical admittance diagrams of the structure without and with the AR layers at 400 nm (first row), 500 nm (second row), and 600 nm (third row). The intersection of the two black dashed lines represents the point (1,0).
3. Results and Discussion
The spectral performance of the sample obtained by design and preparation can be calculated intuitively to obtain the color performance. The reflection spectra at normal incidence are obtained with a thin-film measurement instrument (UV-1900I). Figure 3(a) provides the calculated reflection spectra (red) of the designed structure at normal incidence, presenting a good agreement with measured reflection spectra (blue). The reflection spectra obtained with and without AR layers are shown in Fig. 3(b). It can also be seen from the spectrum that the reflection below 600 nm is greatly suppressed, and a peak reflection of 91% is achieved at the longer wavelengths, both of which are conducive to the production of high-purity red color. The color coordinates are evaluated according to the calculated and measured reflections under the standard Illuminant D65 and described on the CIE 1931 chromaticity diagram, as shown in Figs. 3(c) and 3(d). The intersection of two solid black lines in the figure represents the standard red (0.64, 0.33) used in liquid crystal displays (LCDs). As can be seen from the figure, the color coordinates of both the simulated (0.63, 0.34) and the measured (0.62, 0.32) are very close to the standard red used in LCDs. The experimental results show that the red color we designed is of high purity.
Figure 3.(a) Calculated (red) and measured (blue) reflection spectra of the proposed device at normal incidence. (b) Calculated spectral reflectance curves with (red line) and without (blue line) the AR layers. (c), (d) Illustration of color coordinates calculated from the reflection spectra in (a) on the CIE 1931 chromaticity diagram.
The high color purity is the result of the combination of AR layers and the absorption properties of these materials. Traditional single-layer AR coating often uses metal oxide materials, such as
SiO is used in the double-layer AR coatings structure. In addition to cooperating with
Figure 4.(a) Absorption spectrum of Ag (black), a-Si (red), and SiO (blue). (b) Absorption spectrum of whole device. (c) Reflection spectra at different incidence angles. (d), (e) Simulated and measured angle-insensitive reflection spectrum. (f) Pictures of the sample observed from different angles.
Figures 4(c), 4(d), and 4(e) show the reflection spectrum of the simulated structure and the actual manufactured sample where angle and wavelength vary. It can be seen from the figure that as the angle increases, the spectral shift of the structure is small. The designed structure can maintain the red appearance in the range of 0° to 60°. The picture of the sample prepared in the laboratory is shown in the Fig. 4(f), and the proposed structure has good angle properties.
4. Conclusion
All in all, we have proposed red structural color with high color purity and high brightness based on the theory of absorption and light interference. High reflection is guaranteed by providing both steep reflections (about 91% at peak) beyond 600 nm and low absorption at the longer wavelengths. During the deposition process, the roughness of the Ag layer is reduced by controlling the deposition mode and temperature, which further enhances the reflection at the longer wavelengths. By using sufficient absorption to suppress the reflection below 600 nm and the AR layers, high color purity of the structure is ensured. The proposed structure is also angle-insensitive and can maintain the red appearance within 60°. The thin films were prepared by electron beam evaporation and combined ion-assisted deposition technology. Only the deposition is required for the device fabrication, thus providing the possibility of mass production at lower cost. The design in this paper may be useful for a variety of applications including color displays, image sensors, and so on.
References
[1] K. Kumar, H. Duan, R. S. Hegde, S. C. W. Koh, J. N. Wei, J. K. W. Yang. Printing colour at the optical diffraction limit. Nat. Nanotechnol., 7, 557(2012).
[2] J. P. Vigneron, J.-F. Colomer, N. Vigneron, V. Lousse. Natural layer-by-layer photonic structure in the squamae of Hoplia coerulea (Coleoptera). Phys. Rev. E, 72, 061904(2005).
[3] J. Jang, T. Badloe, Y. Yang, T. Lee, J. Mun, J. Rho. Spectral modulation through the hybridization of Mie-scatterers and quasi-guided mode resonances: realizing full and gradients of structural color. ACS Nano, 14, 15317(2020).
[4] A. C. Arsenault, D. P. Puzzo, I. Manners, G. A. Ozin. Photonic-crystal full-colour displays. Nat. Photon., 1, 468(2007).
[5] C. Ji, K.-T. Lee, T. Xu, J. Zhou, H. J. Park, L. J. Guo. Engineering light at the nanoscale: structural color filters and broadband perfect absorbers. Adv. Opt. Mater., 5, 1700368(2017).
[6] H. Zhou, J. Xu, X. Liu, H. Zhang, D. Wang, Z. Chen, D. Zhang, T. Fan. Bio-inspired photonic materials: prototypes and structural effect designs for applications in solar energy manipulation. Adv. Funct. Mater., 28, 1705309(2018).
[7] P. B. Catrysse, W. Suh, S. Fan, M. Peeters. One-mode model for patterned metal layers inside integrated color pixels. Opt. Lett., 29, 974(2004).
[8] K. Chung, S. Yu, C.-J. Heo, J. W. Shim, S.-M. Yang, M. G. Han, H.-S. Lee, Y. Jin, S. Y. Lee, N. Park, J. H. Shin. Flexible, angle-independent, structural color reflectors inspired by morpho butterfly wings. Adv. Mater., 24, 2375(2012).
[9] J. Hong, E. Chan, T. Chang, T.-C. Fung, B. Hong, C. Kim, J. Ma, Y. Pan, R. Van Lier, S.-G. Wang, B. Wen, L. Zhou. Continuous color reflective displays using interferometric absorption. Optica, 2, 589(2015).
[10] S. Yokogawa, S. P. Burgos, H. A. Atwater. Plasmonic color filters for CMOS image sensor applications. Nano Lett., 12, 4349(2012).
[11] T. Teng, C. Sun. Integrating backlight with color-filter-free panel for enhancing performance of LCD. IEEE Photon. J., 12, 7000116(2020).
[12] Y.-T. Yoon, H.-S. Lee, S.-S. Lee, S. H. Kim, J.-D. Park, K.-D. Lee. Color filter incorporating a subwavelength patterned grating in poly silicon. Opt. Express, 16, 2374(2008).
[13] S. Jiang, Y. Hu, H. Wu, Y. Zhang, Y. Zhang, Y. Wang, Y. Zhang, W. Zhu, J. Li, D. Wu, J. Chu. Multifunctional Janus microplates arrays actuated by magnetic fields for water/light switches and bio-inspired assimilatory coloration. Adv. Mater., 31, 1807507(2019).
[14] H. J. Park, T. Xu, J. Y. Lee, A. Ledbetter, L. J. Guo. Photonic color filters integrated with organic solar cells for energy harvesting. ACS Nano, 5, 7055(2011).
[15] C. Yang, W. Shen, Y. Zhang, K. Li, X. Fang, X. Zhang, X. Liu. Compact multilayer film structure for angle insensitive color filtering. Sci. Rep., 5, 9285(2015).
[16] C. Yang, W. Shen, Y. Zhang, H. Peng, X. Zhang, X. Liu. Design and simulation of omnidirectional reflective color filters based on metal-dielectric-metal structure. Opt. Express, 22, 11384(2014).
[17] Y. Hu, H. Yuan, S. Liu, J. Ni, J. Chu. Chiral assemblies of laser-printed micropillars directed by asymmetrical capillary force. Adv. Mater., 2002356(2020).
[18] Y.-K. R. Wu, A. E. Hollowell, C. Zhang, L. J. Guo. Angle-insensitive structural colours based on metallic nanocavities and coloured pixels beyond the diffraction limit. Sci. Rep., 3, 1194(2013).
[19] J. Chu, J. Wang, J. Wang, X. Liu, Y. Zhang, L. Shi, J. Zi. Structural-colored silk based on Ti-Si bilayer. Chin. Opt. Lett., 19, 051601(2021).
[20] Z. Shao, K. Wu, J. Chen. All-optical inverter based on carbon nanotube-polyvinyl alcohol thin film. Chin. Opt. Lett., 18, 060603(2020).
[21] M. A. Kats, S. J. Byrnes, R. Blanchard, M. Kolle, P. Genevet, J. Aizenberg, F. Capasso. Enhancement of absorption and color contrast in ultra-thin highly absorbing optical coatings. Appl. Phys. Lett., 103, 101104(2013).
[22] A. S. Rana, M. Zubair, M. S. Anwar, M. Saleem, M. Q. Mehmood. Engineering the absorption spectra of thin film multilayer absorbers for enhanced color purity in CMY color filters. Opt. Mater. Express, 10, 268(2020).
[23] L. Zhao, H. Liu, Z. He, S. Dong. Wide-angle polarization-controllable structure color based on metamaterial resonators with polarized multiband absorption peaks. IEEE Photon. J., 10, 2700410(2018).
[24] M. A. Kats, R. Blanchard, P. Genevet, F. Capasso. Nanometre optical coatings based on strong interference effects in highly absorbing media. Nat. Mater., 12, 20(2013).
[25] C. Ji, K.-T. Lee, L. J. Guo. High-color-purity, angle-invariant, and bidirectional structural colors based on higher-order resonances. Opt. Lett., 44, 86(2019).
[26] K.-T. Lee, C. Ji, D. Banerjee, L. J. Guo. Angular- and polarization-independent structural colors based on 1D photonic crystals. Laser Photon. Rev., 9, 354(2015).
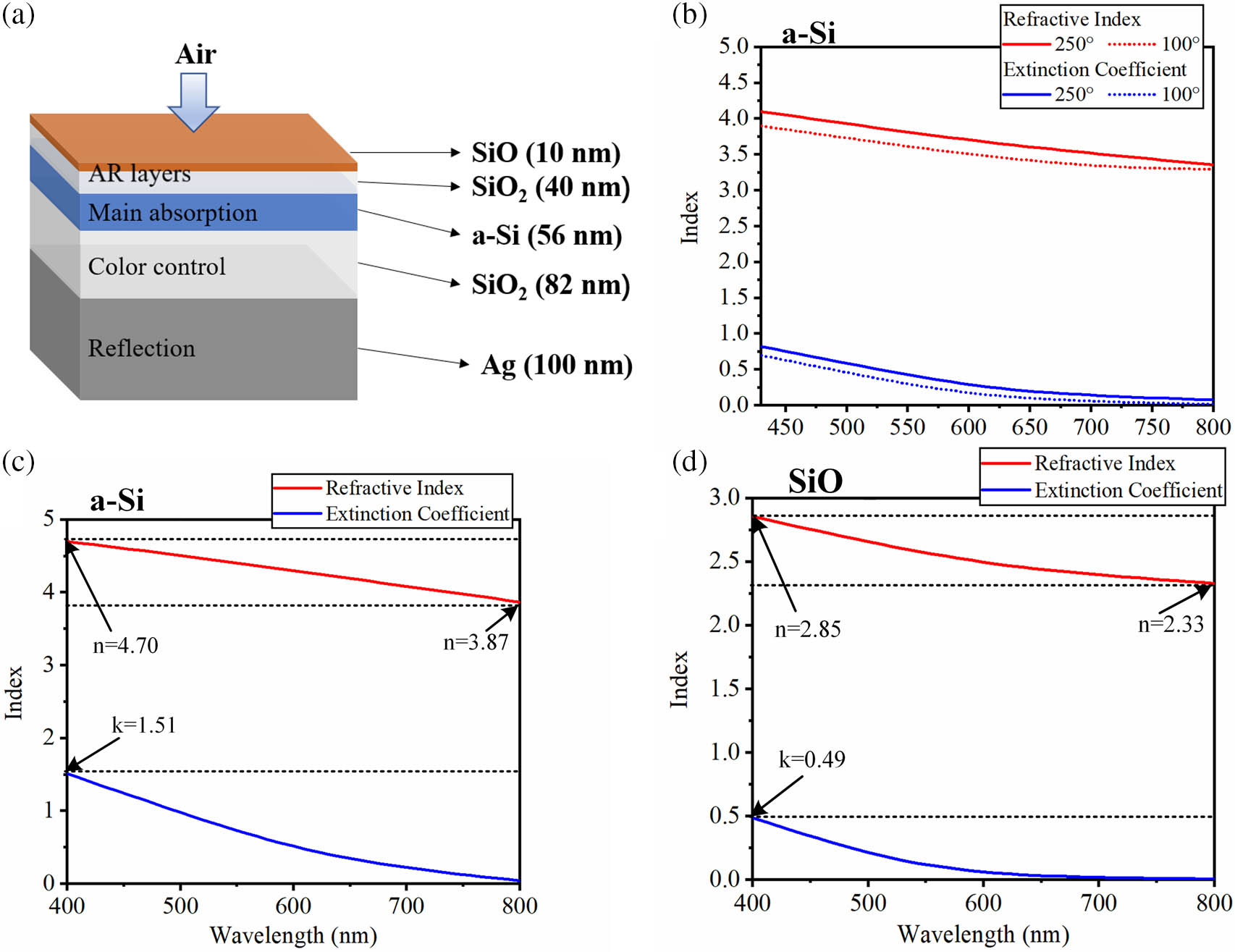
Set citation alerts for the article
Please enter your email address