Abstract
Metamaterials composed of metallic antennae arrays are used as they possess extraordinary optical transmission (EOT) in the terahertz (THz) region, whereby a giant forward light propagation can be created using constructive interference of tunneling surface plasmonic waves. However, numerous applications of THz meta-devices demand an active manipulation of the THz beam in free space. Although some studies have been carried out to control the EOT for the THz region, few of these are based upon electrical modulation of the EOT phenomenon, and novel strategies are required for actively and dynamically reconfigurable EOT meta-devices. In this work, we experimentally present that the EOT resonance can be coupled to optically reconfigurable chalcogenide metamaterials which offers a reversible all-optical control of the THz light. A modulation efficiency of 88% in transmission at 0.85 THz is experimentally observed using the EOT metamaterials, which is composed of a gold (Au) circular aperture array sitting on a non-volatile chalcogenide phase change material (Ge2Sb2Te5) film. This comes up with a robust and ultrafast reconfigurable EOT over 20 times of switching, excited by a nanosecond pulsed laser. The measured data have a good agreement with finite-element-method numerical simulation. This work promises THz modulators with significant on/off ratios and fast speeds.Metamaterials composed of metallic antennae arrays are used as they possess extraordinary optical transmission (EOT) in the terahertz (THz) region, whereby a giant forward light propagation can be created using constructive interference of tunneling surface plasmonic waves. However, numerous applications of THz meta-devices demand an active manipulation of the THz beam in free space. Although some studies have been carried out to control the EOT for the THz region, few of these are based upon electrical modulation of the EOT phenomenon, and novel strategies are required for actively and dynamically reconfigurable EOT meta-devices. In this work, we experimentally present that the EOT resonance can be coupled to optically reconfigurable chalcogenide metamaterials which offers a reversible all-optical control of the THz light. A modulation efficiency of 88% in transmission at 0.85 THz is experimentally observed using the EOT metamaterials, which is composed of a gold (Au) circular aperture array sitting on a non-volatile chalcogenide phase change material (Ge2Sb2Te5) film. This comes up with a robust and ultrafast reconfigurable EOT over 20 times of switching, excited by a nanosecond pulsed laser. The measured data have a good agreement with finite-element-method numerical simulation. This work promises THz modulators with significant on/off ratios and fast speeds.Introduction
Terahertz (THz) electromagnetic (EM) waves residing in the regime between infrared and microwave spectra have attracted intense attention due to their appealing advantages of excellent transparency, low photon energy, and high spectral resolution. Many works have exploited the interferences of THz waves with matter, for which promising applications include security inspection, non-destructive evaluation, medical imaging, chemical identification, and high-speed wireless communications1-4. Yet, a complete exploration of constructive applications over the THz regime is limited owing to the unavailability of appropriate responses in the THz region for numerous naturally present materials5. Metamaterials are artificially structured materials whose EM properties are mainly decided by the meta-atom profile, enabling them to produce novel EM functions that are not present in natural materials, such as cloaking, negative refractive index, and superlens6-11. Moreover, the light-matter interference can be considerably enhanced since the meta-atom offers intense confinement of EM-fields in a subwavelength area over almost the whole EM-spectrum. Such metamaterials can be used to develop various novel THz devices, which are traditionally hard to obtain owing to the lack of proper sources and sensors12, 13. Notably, metamaterials induced extraordinary optical transmission (EOT) operating in the THz region has become an extremely active research area. It results in both exploring new methods of improving optical transmission and applying it to detecting, imaging, and nonlinear enhancement, among others14-16. Moreover, the capability of modulating the EOT effect is appealing for practical applications. Recently, a few efforts have been made in controlling the EOT effect in the THz region using metamaterials incorporated with different active mediums such as graphene17, 18 and vanadium dioxide (VO2)19, 20. Graphene is a promising platform for THz modulators since it can obtain the electrically engineered density of states for intraband transition in the THz region21-23. Nevertheless, the one-atomic-layer thickness and low free carrier density of graphene limit the efficiency (i.e., extinction ratio) of these THz modulators, where a non-resonant nature of the intraband absorption exists24, 25. Vanadium dioxide (VO2) is also a promising candidate for tuning the EOT response from subwavelength apertures in a metal layer19, 26 due to its phase transition from insulator to metal27, 28. However, the practical suitability of VO2 for terahertz EOT meta-devices is hampered by its volatility, complicated fabrication, and limitations of multilevel response and reversible phase transition29. Many works have demonstrated metamaterials based on the various active materials for actively controlling the EOT effect. Yet, to our knowledge, no EOT meta-devices presented to date have a combination of an ultrafast speed, a considerable modulation efficiency, nonvolatility, and repeatable reversible switching. It is, however, a highly important technique that may take a big stride forward towards practical applications.
An exciting new opportunity is suggested by recent developments in the chalcogenide phase change materials (PCMs)30, 31. Chalcogenide PCMs such as Ge-Sb-Te alloys undergo phase transitions facilitated by nucleation dynamics32; therefore, by continuously crystallizing the Ge-Sb-Te alloys, an analogue response can be obtained instead of binary switching states. Note that these analogue states are non-volatile; energy is only needed for the switching activity and not for maintaining an individual state33, 34. Moreover, the Ge-Sb-Te alloys possess loads of desirable transiting features, such as short switching periods and high cyclability35. Thus this fuels the epoch of rewritable optical data storage and memory36, 37. Furthermore, the fast, reversible, and non-volatile amorphous-to-crystalline switching in the most studied Ge-Sb-Te alloy, Ge2Sb2Te5 (GST225), makes it ideal for reconfigurable photonic devices38-40. Very recently, nanostructured GST225 has been exploited to achieve specific switching properties41-43. The usability of GST225 for the different kinds of photonic devices is because that the dielectric properties of GST225 undergo a significant variation during its structural state change in the infrared region44, 45. Since the radical contrast of optical property of the chalcogenide PCMs ranges from the IR to THz regions, it is promising for tunable THz meta-devices. The chalcogenide PCM of GeTe has been studied previously for actively controlling the THz wave with nonvolatility46. Subsequently, a very recent work showed that THz chalcogenide metamaterials with a broken symmetry could realise a non-reversible tuning of Fano resonance by thermal annealing systems47. These works show that the chalcogenide PCMs can be valuable materials for obtaining actively tunable metamaterials in the THz regime. Yet, the versatile nature of the chalcogenide PCMs and their potential in the realisation of dynamically reconfigurable THz wave modulations have remained mostly undiscovered.
In this paper, we present an approach for the realization of dynamical, scalable, non-volatile, and repeatedly reconfigurable meta-devices to manipulate the EOT effect in the THz region. This metamaterial is composed of an array of subwavelength gold (Au) holes that are fabricated on the GST225 switchable laminate. The EOT resonance results from the coupling of the THz wave with plasmons—electronic excitations—on the surface of the Au holes array. By optically changing the GST225 conductivity via an amorphous—crystalline state change, we accomplish switching on/off of the EOT with a modulation efficiency of intensity as high as 88%. Furthermore, we experimentally show that such metamaterials can provide a very robust reconfigurable EOT effect over 20 times of transition. With the benefits of multiple-time reversible switching, high on/off ratios, simple geometry, and lightweight, our proposed meta-devices may be suitable for applications in diverse fields of THz sensing, communications, imaging, and so on.
Results and discussion
Design and fabrication
Figure 1(a) schematically shows the GST225-based THz modulator. The EOT THz metamaterials composed of Au holes array are placed above the 0.1 μm thick GST225 layer sitting on the silicon (Si) substrate. The pitch of the metamaterials is p = 100 μm, while the diameter and depth of the Au holes are d = 60 μm and hAu = 200 nm, respectively. To avoid interlayer diffusion and interfacial reactions, a 5 nm thick silicon nitride (Si3N4) diffusion barrier was deposited between the Au holes and GST225 laminate48. The finite element method (FEM) is employed to solve Maxwell's equations for the metamaterial based on the amorphous GST225. We use the measured conductivity (σ) of the GST225 film at room temperature (see a black line in Fig. 1(d)). The Au was modelled as a lossy metal with a conductivity of 4.1×107 S/m. All the metamaterials are modelled under normal plane wave illumination and the wave vector pointing towards the +z direction, see Fig. 1(a). In Fig. 1(b), we numerically explored the dependence of the diameter and depth of the Au hole on the transmission of the chalcogenide metamaterials with amorphous state. In the top panel, we simulated the transmission for hAu = 200 nm at the different d. As was seen, the peak transmission increased with d and tended to reduce when d > 60 μm. In the bottom panel, we numerically presented the transmission spectrum of the EOT metamaterials by varying the hAu from 50 nm to 300 nm at d = 60 μm. As was seen, the peak transmission increased with the hAu while it was maintained for hAu ≥ 100 nm. It is because that the transmission spectrum of the EOT metamaterials was independent of the Au film thickness when it is larger than the skin depth in the Au49, 50. Thus, herein the diameter and height of the Au holes were determined at d = 60 μm and hAu = 200 nm. The simplicity of this EOT metamaterial design is promising for applications in the THz integrated devices.
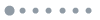
Figure 1.Configuration of the EOT metamaterials for the THz region. (a) Schematic of the all-optical, reconfigurable, non-volatile phase-change metamaterials induced EOT switch: single nanosecond pulsed laser transits a 100 nm thick GST225 film reversibly between the amorphous and crystalline states. (b) The FEM simulated transmission spectra of the chalcogenide metamaterials with the amorphous state at the various diameter of d = 40, 60, and 80 μm (top panel) and various heights of hAu = 50, 80, 100, 200, and 300 nm (bottom panel). (c) Optical microscope (top panel) and FIB cross-sections (bottom panel) images of the EOT metamaterials. The geometrical parameters of the subwavelength holes array are p = 100 μm, d = 60 μm, respectively; the thicknesses of the Au and GST225 layers are hAu = 0.2 μm and hGST= 0.1 μm, respectively. (d) The temperature-dependent optical conductivity (σ) of the 100 nm thick GST225 film. (e) The behavior of resonant transition in the metamaterials: numerical simulated (top panel) and experimental measured (bottom panel) transmission spectra at the various temperatures ranging from 25°C to 300°C. The reduction of the peak intensity can be experimentally and theoretically observed by increasing the temperature. (f) The modulation efficiency against the annealing temperature varied from 25 °C to 300 °C.
The EOT originates from excitation of the surface Bloch mode (SBM). Such a resonant SBM can enhance the field at the Au surface and, thus, the evanescent funneling of THz wave through the subwavelength holes array51, 52. Namely, the EOT was created by the multiple scatterings of flat-interface surface waves launched by each Au hole in the array53. Two different surface waves are involved, the surface plasmon polariton and quasi-cylindrical wave, which appear with a fixed mixing ratio to form a hybrid wave54, 55. Particularly, in the EOT phenomenon, incident light is scattered by the Au subwavelength holes array into surface plasmons on the top surface of the Au. Subsequently, the surface plasmons tunnel through the Au holes array and excite surface plasmons on the bottom surface of the Au. Then, the surface plasmon on the bottom Au surface re-emits into free space, leading to a transmitted diffraction peak with a high peak intensity at the EOT resonance frequency56-58. The subwavelength Au holes play an essential role in the EOT effect through optical coupling between the surface plasmons on the top and bottom Au surfaces. Consequently, the total electric (E-) field is significantly enhanced around and inside the Au subwavelength holes, exciting the EOT phenomenon.
In our proposed modulator, GST225 film is placed below the Au holes to engineer the EOT resonant coupling. This is achieved by modulating the conductivity of GST225 via its phase transition between amorphous and crystalline. For the amorphous state, the GST225 film possesses a minimal conductivity and is transparent at the operating frequency, allowing for a high transmission of THz wave at the EOT resonance. For the crystalline state, the conductivity of GST225 significantly increases to block the wave transmission at the EOT resonance. Thus, an intense suppression of EOT appears. To illustrate this tuning mechanism, we fabricated the subwavelength Au nanoholes array on the GST225 membrane, and the optical microscope image of the structure was presented in the top panel of Fig. 1(c). A 100 nm thick GST225 laminate is first fabricated onto a 1 mm thick silicon (Si) substrate. The Au holes array is made using standard photolithography techniques and electron beam deposition of 200 nm of Au, followed by a lift-off procedure. A flowchart of the entire fabrication process is schematically illustrated in Supplementary information
Fig. S1. The sample has a relatively large array area of 4 cm× 4 cm so that a focused THz beam can fit. A focused ion beam (FIB) cross section of the metamaterials, which is presented in bottom panel of Fig. 1(c), can be employed to find the accurate thickness of each layer of the metamaterials. As was seen, the measured thicknesses of Au, Si3N4, and GST225 films are 200, 5, and 100 nm, respectively.
The optical conductivity (σ) of 100 nm thick GST225 film in the THz region is extracted from the transmitted THz time signal through the GST225 film with the Si substrate as a reference. Fig. 1(d) presents the temperature dependent σ. By thermally crystallizing the as-deposited (AD) amorphous (AM) GST225 film, the σ possesses a pronounced contrast and is weakly dispersive over the THz region. A large increase in the σ is found with a three increasing order as transiting the GST225 state from AD-AM to crystalline (CR). For example, the σ of AD-AM GST225 film is ~108 S/m (black line) which is boosted to ~321868 S/m for 300 °C annealed CR film (red line). Such a remarkable change in the σbetween the AD-AM (black line) and CR (red line) states can switch the EOT response “on/off” at a fixed THz frequency. The large difference of σ in GST225 originates from bonding switching between the predominantly covalent bonds in the AM state and resonant bonds in the CR state30, 40. Namely, the CR- GST225 has an extremely large σ, resulting from highly polarisable delocalizedp-orbital resonant bonding. The AM-GST225 that is built from the predominantly covalent bonds has a much lower σ. Note that the GST225 film is gradually crystallised, which can form an intermediate state exhibiting regions of both amorphous and crystalline phases59. By controlling the annealing time and temperature, the crystalline fraction in the AD-AM GST225 film can be finely modified, leading to a gradual variation in the σ. Such an attractive feature can offer a more continuous modulation of the EOT effect in the proposed THz metamaterials. To show this, the measured σ of the GST225 film at the different heating temperatures is also demonstrated in Fig. 1(d). As can be seen, the σincreases with the temperature in the spectral range from 0.2 to 1.8 THz. Note, the largest change in the σ appears when increasing the temperature from 200 to 300 °C. Importantly, these intermediate states of GST225 are non-volatile. Therefore, they are very stable at room temperature, and the thermal energy is only required for switching the structural state, not for maintaining a certain state. This makes the continuously tunable THz EOT meta-device appealing from a green technology perspective.
The FEM simulated transmission spectra under the different temperatures are shown in the top panel of Fig. 1(e). We use the measured temperature-dependent conductivity (σ) of the GST225 film that is shown in Fig. 1(d). The metamaterial geometry is set to that measured using the optical microscope and FIB cross-section images presented in Fig. 1(c). A decrease of the resonant peak occurs by increasing the temperature. The transmission (T) of the fabricated THz chalcogenide metamaterials under the different temperatures, from 25 °C to 300 °C are measured by THz time-domain spectroscopy (THz-TDS) system. The THz time-domain data are temporally windowed to remove the effect of multiple reflectances within the Si substrate. The measured transmission spectra are shown in the bottom panel of Fig. 1(e). The transmission spectra are polarisation independent due to the structural symmetry. As can be seen, the peak transmission decreases with the temperature, while the central frequency remained almost the same at different temperatures. For the AD-AM state, the EOT resonance is measured at f1=0.85 THz and reaches the maximum value of T = 0.63 (black line) due to the tiny σ of GST225 film. Note that starting with the TC =250 °C measurement (purple line), the GST225 film is crystallized to obtain a high σ, thus demonstrating a metallic behavior. This is manifest by the radical variation in the transmission spectra between the measurements taken at 200 °C (cyan line) and 250 °C (purple line). When thermally annealed to 300 °C, the EOT resonance is entirely turned off (red line). The numerically calculated transmission spectra under the different temperatures have a good agreement with the measured ones. The FEM simulations (top panel) slightly differ from the experimental measurements (bottom panel) in a few ways. The simulated modulation of EOT intensity is higher than the experimental one, and the bandwidth of the measured resonance is significantly wider. These characteristics are owing to certain factors that differentiate simulations from measurements. The incident light is lightened by a Cassegrain-type microscope objective lens possessing a numerical aperture (NA) of 0.58, showing the angle of incident light ranges from –35° to +35°. Such a wide angular distribution of incident light leads to a lower transmission in the EOT resonance and a wider transmission spectrum compared to the simulated one. This is because the EOT resonance is strongly associated with the incident angle. Besides, in the model, we ignore the fabrication imperfections, surface roughness and native oxides. We presume this simplification causes the slight broadening of the experimentally measured spectra and the reduction of the modulation efficiency compared to the numerical calculation. We then demonstrate how the metamaterial transmission spectra can be actively controlled by transiting the state of the GST225 via the temperature variation. This capability to cover a portion of the THz region by gradually crystallising the GST225, is the core of the THz modulator.
In Fig. 1(f), we have evaluated the modulation performance of the meta-devices. Herein, the modulation efficiency (ζm ) in the transmission is defined by ζm= 1–T /Tmax, where transmission T is dependent on the temperature, and Tmax is the peak transmission for the AD-AM metamaterials that exhibit the most substantial transmitted intensity at the resonant frequency. The ζm increases with the temperature. For instance, at the room temperature of 25 °C, the modulation efficiency of the metamaterials is ζm = 0, while the EOT metamaterials can achieve ζm = 88% at the temperature of 300 °C. Therefore, the EOT metamaterials can possess a significant modulation in transmission by switching the state of the GST225 between amorphous and crystalline states. Modulation efficiency is a crucial parameter for evaluating the performance of the modulators. In the last decade, the THz modulators have developed rapidly, and the modulation efficiency has increased from 40% to nearly 90%60. Our proposed chalcogenide metamaterials with a high modulation efficiency of 88% may build up a platform for developing new kinds of THz modulators (i.e., compressing sensor modulator and on-chip modulator)61, which provides an extraordinary function for manipulating THz waves.
Experimental realization of reconfigurable EOT
The GST225 film exhibits crystallization and melting temperatures of TC = 250 °C and TM = 600 °C, respectively. It has been highly developed as a platform for efficient, non-volatile, prompt, reproducible state switching62, 63. Herein, the alternation between the amorphous and crystalline structural phases offers a significant change in the σ of GST225 film, which subsequently modulates the EOT resonant intensity. The amorphisation of GST225 can be experimentally realised by the illumination of a nanosecond (ns) pulsed laser, while the recrystallisation of GST225 can be realised by the hot plate64, 65. In Fig. 2(a), we schematically show the phase change metamaterials treatment. First, the AD-AM GST225 film is crystallised by heating the metamaterials for 30 mins at TC=250 °C on a hot-plate in a flowing Ar atmosphere. To reversibly transit the structural state of the GST225 film from CR to MQ-AM, the crystal lattice is melted and then quenched into the AM state (room temperature) under a rate of 109~1010 K/s that prevents recrystallisation of the atomic structure66, 67. Ultrashort laser Joule pulses can provide such a quench rate68, which is employed to switch the 100 nm thick GST225 film from the CR to AM states. Particularly, to amorphise the crystalline GST225 laminate, a single 5 ns laser pulse makes the local temperature momentarily increase above 600 °C to melt the GST225. A fast-cooling processing is subsequently performed to quench the melted layer into the AM phase. To recrystallise the MQ-AM GST225 layer, a hot plate is used to hold the GST225 film above TC= 250 °C but below TM= 600 °C. The phase transition (amorphous to crystalline) of GST225 can also be electrically achieved by integrating a bottom metallic heater to the metasurfaces, as schematically shown in Supplementary information
Fig. S2.
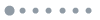
Figure 2.In sequence processing for the reversible state change. (a) Schematic of the reversible state change of the GST225 layer hybridised with an EOT metamaterials: the AD-AM GST225 is initially heated above TC=250 °C to switch to the CR-GST225 via a hot plate. A single ns pulsed laser is transited to thermally anneal the CR-GST225 layer above TM=600 °C that reamorphises the CR-GST225. Consequent quenching leads to the MQ-AM GST225. A temperature above TC=250 °C but below TM=600 °C is needed to recrystallise the MQ-AM GST225, which is achieved by using a hot plate. (b) The σ of 100 nm thick GST225 film at the various structural states of the as-deposited amorphous (AD-AM, black line), crystalline (CR, red line), melt quenched amorphous (MQ-AM, grey line), and re-crystallised (R-CR, orange line) over a spectral range of 0.2-1.8 THz. Experimental realisation of reversibly tunable EOT effect: the THz-TDS measurement of the transmission spectra of the chalcogenide metamaterials with the various structural phases of (c) AD-AM, CR and (d) MQ-AM, and R-CR.
Figure 2(b) shows the σ of a 100 nm thick monolithic GST225 layer for the AD-AM (black line), CR (red line), MQ-AM (grey line), and re-crystallised (R-CR, orange line) structural phases. Note, the spectra of the optical properties (σ) for AD-AM and MQ-AM GST225, as well as for CR and R-CR GST225, are almost identical. It confirms that the GST225 phase transition can be reversible, and the material after the ultrashort pulsed laser-induced change resembles the initial states after hot-plate annealing. In Fig. 2(c)–2(d), we demonstrate experimentally a reconfigurable metamaterial modulator of EOT operating at the THz frequency using a reversible phase transition between the AM and CR in the GST225 film. The transmission spectra are divided into two sections to distinguish between the metamaterial treatments: a comparison between the AD-AM (black line) and thermally annealed CR (red line) states is presented in Fig. 2(c), and the comparison between the MQ-AM (grey line) and R-CR (orange line) states after ns laser-induced phase transition is shown in Fig. 2(d). When the GST225 is in the AD-AM and MQ-AM states, the EOT resonant peak with a transmission magnitude of 0.63 at f1=0.85 THz occurs. When the GST225 is crystallised by thermal annealing (CR and R-CR), the EOT resonance vanishes. This is because the σ of the CR- GST225 is much larger than that of the AM-GST225 (Fig. 2(b)), which increases the loss to decrease the peak intensity and broaden the bandwidth of the EOT phenomenon. This produces a tremendous modulation efficiency at f1=0.85 THz. The active transmission engineering in the meta-device possesses a clear advantage over the previously shown passive THz EOT metamaterials.
Resonant modes in EOT
The origin of the EOT effect is based upon the strong enhancement of the total electrical field intensity (
) inside the Au subwavelength holes array. In order to observe this origin, it is helpful to investigate the distributions of the total E-field intensities of the metamaterials at the different temperatures along the x-z cross-section plane. Fig. 3 presents the E-field distributions at the resonant frequencies of 0.87 THz corresponding to the various temperatures of 25 °C, 150 °C, 200 °C, and 300 °C, respectively. In the profile, the colour represents the magnitude of the E-field intensities. The THz wave is incident from the top of the metamaterials. It demonstrates that the EOT effect can be interpreted by a cavity resonance. The total E-field intensity distribution for the amorphous state is shown in Fig. 3(a). It clearly shows that the total E-field can couple in the GST225 film and is intensely enhanced around and inside the hole region, which is a feature of a typical EOT effect. In Fig. 3(b)–3(d), the total E-field intensity distributions atf = 0.87 THz for the various temperatures of 150 °C, 200 °C, and 300 °C are explored. It can be seen that with an increase of the temperature, the EOT resonance is largely damped and hence suppresses the total E-field inside the holes.
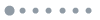
Figure 3.Numerical simulation of total E- field distribution along the x-z plane of the metamaterials at the various temperatures of (a) 25 °C, (b) 150 °C, (c) 200 °C, and (d) 300 °C.
Repeatedly rewritable experiments
To examine the repeatability of optical switching of the EOT metamaterial, we repeatedly performed rewritable experiments. Fig. 4(a) experimentally illustrates the transmission spectra of the EOT metamaterials for twenty transition times. As can be seen, the metamaterials give rise to a very efficient reconfigurable EOT effect over many times of switching, taking advantage of a diffusion barrier of Si3N4 between the Au holes and the GST225 film. Fig. 4(b) presents the values of the peak transmission for amorphous (black dots) and crystalline (red dots) states with twenty switching times. Herein, the amorphous GST225 film can be crystallised by thermally annealing the metamaterials for 3 min at T=300 °C on a hotplate. To amorphise the crystalline GST225 film, an ultrafast laser pulse was employed to momentarily increase the temperature of GST225 film above 600 °C and then cool promptly down into the room temperature. The ultrafast laser pulse has a central wavelength of 1064 nm, pulse width of 5 ns, a spot diameter of 1 cm, and peak fluence of 105 mJ/cm2. Such a rewritable experiment was cycled to achieve the repeatable optical switching of the EOT response.
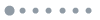
Figure 4.(a) Measured transmission spectra of the EOT chalcogenide metamaterials for twenty switching times. (b) The values of transmission peaks for the amorphous (shown by black dots) and crystalline (indicated by red dots) states with twenty switching times. The morphology (top panel) and cross-sectional (bottom panel) images of the chalcogenide metamaterials (c) before and (d) after 20 transition times.
In the proof-of-principle metamaterials experimentally realised here, the functional GST225 layer typically endures ≈10 switching cycles (amorphous-to-crystalline and backward) before demonstrating the degradation sign. It is induced by degradation of the GST225 due to the formation of voids. Initially, the tiny voids with a few nanometers size were distributed inside the crystalline GST225 layer. After more repeated switching cycles, the sizes of voids have become larger69. This leads to gradual segregation of the elements of Ge, Sb, and Te in the various regions of the device. Such segregation can slowly change the switching features of the device70. Moreover, the atomic contents of Ge, Sb, and Te varied after repeated heating around the voids71, enabling the compositional variations to be closely associated with the void formation in the GST225 layer. These compositional changes may reduce endurance characteristics during the metadevice operations72-74. It is expected that the device robustness can be improved by optimizing the compositions and thicknesses of GST225 film. The melting point temperatures of silicon (Si) and silicon nitride (Si3N4) are TSi = 1410 °C and TSiN = 1990 °C, respectively75, which are much higher than the melting temperatures (TM = 600 °C) of GST225 film. Therefore, the Si and Si3N4 films were not significantly affected after 20 transition times. Figure 4(c) and 4(d) present the FIB images of the chalcogenide metamaterials before and after 20 transition times, where the morphology and side view of the metamaterials were shown in top and bottom panels, respectively. As was observed, the thickness of each layer and morphology of the Au holes array can be maintained during the phase transition of GST225. Moreover, by integrating the GST225 active component into the various functional resonators such as split-ring76, 77, and H-shaped78 resonators, one may also reversibly tune the resonant wavelength of the THz meta-devices. Beyond the reconfigurable EOT phenomenon, the GST225 based metamaterials may also find interesting applications for reconfigurable extraordinary optical diffraction response79, 80.
Conclusions
In conclusion, we have demonstrated a reconfigurable EOT metamaterial for the THz region, realised by ns laser-induced switching of the structural phase of the chalcogenide glass, GST225. The chalcogenide metamaterials provide non-volatile and active controlling of the EOT resonant mode by transiting the state of GST225 between amorphous and crystalline. Such a phase change leads to a large contrast in the σ, changing from 108 S/m to 321868 S/m, which excites the EOT for the amorphous state, whereas suppresses the EOT for the crystalline state, causing a very high modulation efficiency of 88% at 0.85 THz. Importantly, the EOT effect can be reversibly tuned over 20 times of switching. These findings may offer chances in designing an on-chip THz wave modulator with a high on/off ratio, low power, high speed, and tailored operating frequency, opening up a range of state-of-the-art applications for THz imaging, communications, and sensing.
Tun Cao acknowledges support from the National Key Research and Development Program of China (2020YFA0714504, 2019YFA0709100) and LiaoNing Revitalization Talents Program (Grant No. XLYC1807237).
Supplementary information for this paper is available at https://doi.org/10.29026/oes.2022.210010
The authors declare no competing financial interests.
References
[75] Haynes WM, Lide DR, Bruno TJ. CRC Handbook of Chemistry and Physics 95th ed (CRC Press, Boca Raton, 2014).